|
|
|
ABSTRACT |
The aim of the study was to find whether voluntary induced high- and low-frequency peripheral fatigue exhibit specific alteration in surface EMG signal (SEMG) during evoked and maximum voluntary contractions. Ten male students of physical education performed 60 s long stretch-shortening cycle (SSC) exercise with maximal intensity and 30 s long concentric (CON) exercise with maximal intensity. To verify voluntary induced peripheral fatigue, knee torques during low- (T20) and high-frequency electrical stimulation (T100) of relaxed vastus lateralis muscle (VL) were obtained. Contractile properties of the VL were measured with passive twitch and maximal voluntary knee extension test (MVC). Changes in M-waves and SEMG during MVC test were used to evaluate the differences in myoelectrical signals. T100/T20 ratio decreased by 10.9 ± 8.4 % (p < 0.01) after the SSC exercise and increased by 35.9 ± 17.5 % (p < 0.001) after the CON exercise. Significant SEMG changes were observed only after the CON exercise where peak to peak time of the M-waves increased by 9.2 ± 13.3 % (p < 0.06), SEMG amplitude during MVC increased by 32.9 ± 21.6 % (p < 0.001) and SEMG power spectrum median frequency decreased by 11.0 ± 10.5 % (p < 0.05). It is concluded that high frequency fatigue wasn't reflected in SEMG, however the SEMG changes after the CON seemed to reflect metabolic changes due to acidosis. |
Key words:
M-wave, stretch-shortening cycle, electrical stimulation, median frequency.
|
Key
Points
- The SSC exercise induced high-frequency fatigue which was not reflected in any SEMG change.
- The CON exercise induced dominantly low-frequency fatigue where only SEMG during MVC changed
- Muscle fibre membrane excitability was not changed due to low- and high-frequency fatigue but mainly reflected metabolic changes.
- Changes in muscle compound action potential did not follow those changes seen after electrically elicited HF and LF fatigue.
|
High- (HF) and low-frequency (LF) peripheral fatigues are specific to contraction type and intensity of exercise (Jereb and Strojnik, 2001; Strojnik and Komi, 1998; 2000; Tomazin et al., 2008). Special joint torque measuring devices and often unpleasant electrical stimulation were used in the above experiments. However, Jones, 1981 showed that surface EMG (SEMG) might be used for monitoring peripheral fatigue as well, which may provide less demanding diagnostic tool for peripheral fatigue and applicable to many muscles simultaneously. Earlier studies using voluntary movements have already suggested that stretch-shortening cycle (SSC) exercise performed with maximal intensity induce HF fatigue (Jereb and Strojnik, 2001; Strojnik and Komi, 1998; Tomazin et al., 2008), while concentric (CON) exercise with maximal intensity (Jereb and Strojnik, 2003) and SSC exercise with submaximal intensity (Strojnik and Komi, 2000) induced LF fatigue. These experiments showed that at muscle force level HF fatigue has been seen as a selective loss of torque at HF electrical stimulation (100 Hz), whereas LF fatigue as a selective loss of torque at LF electrical stimulation (20 Hz). When HF fatigue was electrically elicited, selective loss of torque has been accompanied with reduction in amplitude of muscle compound action potential (MCAP) (Bigland-Ritchie et al., 1979; Darques and Jammes, 1997; Darques et al., 2003; Metzger and Fitts, 1986), followed by complete blockade of MCAP (M-wave) at the end of HF electrical stimulation (Badier et al., 1999; Sandercock et al., 1985). When LF fatigue was electrically elicited, selective loss of torque has been simultaneously accompanied with increased MCAP at the beginning of stimulation, which was followed by minor reduction and prolongation of MCAP at the end of LF electrical stimulation (Badier et al., 1999; Sandercock et al., 1985). Combined in situ analyses of metabolic and myoelectrical changes associated with electrically induced HF and LF fatigue (Darques et al., 2003) showed that force failure during HF stimulation resulted from an impaired propagation of muscle action potential (AP) with no metabolic involvement, while fatigue with LF stimulation was closely associated with metabolic changes with no alteration of muscle membrane excitability. As seen above, differences in MCAP were substantial after electrically induced HF vs. LF peripheral fatigue (Badier et al., 1999; Darques et al. in 2003; Sandercock et al., 1985). Therefore, fatigue with changes in amplitude of MCAP could be recognized as HF fatigue, while fatigue without MCAP changes as LF fatigue. In line with this, the study of Pasquet et al., 2000 interpreted M-wave changes after slow concentric contractions as HF fatigue. Since there are significant differences in muscle activation between electrically elicited and voluntary muscle contraction (Hennings et al., 2007), it would be possible to expect specific response due to voluntary induced LF and HF fatigue in SEMG in comparison to electrically induced fatigue. LF fatigue during CON with maximum intensity is closely linked with intracellular acidosis and/or Pi accumulation without any altered muscle electrically activity (Darques et al., 2003). Lower intracellular pH after CON exercise due to higher blood lactate production, already seen in the studies by Jereb and Strojnik (2003) and Jereb (1995; 1998), could decrease muscle fibre conducting velocity. This could be reflected in greater SEMG amplitude change after CON rather than after a short SSC exercise (Basmajin and DeLuca, 1985; Luttmann et al., 1998). Similarly, greater frequency shift of SEMG power spectrum towards lower values can be expected after CON exercise than after a short SSC exercise with maximum intensity (Basmajin and DeLuca, 1985; Luttmann et al., 1998). The aim of the present study is to analyze differences in SEMG after two different voluntary exercises that will induce high- and low-frequency fatigue. It is expected that SEMG during voluntary and electrically evoked potentials will be specific to peripheral fatigue type and thus enable differentiation between both.
SubjectsTen male students of physical education (24.2 ± 3.4 years of age, 79.3 ± 2.9 kg, 1.79 ± 0.04 m) volunteered for the study. At the time of the experiment, none of them was involved in any intensive sports training. The study was approved by the National Committee for Medical Ethics and was conducted according the Declaration of Helsinki.
Experimental designThe experiment was performed in two parts each induced one fatigue workout. The rest period between parts was exactly one week. The protocol was the same during both parts of the experiment. The subjects warmed up by stepping on a 20-cm-high bench for 6 minutes with a frequency of 0.5 Hz, exchanging leg every minute. After the warm up, the initial measurements were performed in the following order: single supramaximal electrical impulse delivered to the femoral nerve to induce M-wave, three single supramaximal electrical impulse delivered to the relaxed vastusl lateralis muscle (VL) to induce twitch, HF and LF electrical stimulation (ES) delivered directly to the relaxed VL, followed by steady maximum voluntary isometric knee extension. The same measurements in the same order were performed again exactly 60 s after the fatiguing workout. Jereb and Strojnik (2003) demonstrated that passive twitch characteristics of VL muscle returned to initial values already 3-min after hopping with maximal intensity for 60-s, the same was true for HF torque. In some other experiments (electrical stimulation, intensive breathing) HF fatigue recovery times may be inside 15 - 30 minutes (Babcock et al., 1998; Metzger and Fitts, 1986; Stokes et al., 1989).
Fatigue workoutIn the first part of the experiment, the subjects performed a SSC exercise (60-s of hopping with maximum intensity - maximum height and short contact time). A CON exercise (30-s of cycling with maximum intensity) was performed a week later. Both activities had already been proved to induce a different type of peripheral fatigue (Jereb and Strojnik, 2001; 2003). The SSC exercise was exactly controlled by timer and was performed on the force platform (model 9278, Kistler, Winterthur, Switzerland). Data were sampled with frequency of 1000 Hz. The CON exercise was performed on the cycle-ergometer (model 818E, Monark, Vansbro, Sweden) against resistance set to 7.5 % of the body mass (Jereb and Strojnik, 2003). Duration and power during the cycling test were controlled with the POWERTM (Sports Medicine Industries Inc, St Cloud, Minnesota).
Electrical stimulationDuring the measurements, the subjects sat in an isometric knee extension torque-measuring device. The distal part of the right shank was fixed to the force transducer with a constant lever arm to the knee joint axis. The knee joint angle was set to 60°. A force transducer (MES, Maribor, Slovenia) with a range of 0 to 5000 N was used. Data were sampled at 1 kHz using a 12 bit AD converter (Burr-Brown, Tucson, Arizona). For direct muscle stimulation, self-adhering neurostimulation electrodes (5x5 cm, Axelgaard, Fallbrook, CA) were placed over the VL muscle. The distal electrode was placed over the distal part of the muscle belly and the proximal electrode over the middle part of the muscle belly. The electrodes remained fixed during the entire duration of the experimental protocol. For the nerve stimulation, the first electrode (1 cm in diameter) was placed over the femoral nerve distally from inguinal ligament and the second one (5x5 cm) was placed over the great trochanter of the stimulated leg. Each pair of electrodes was connected to a custom built computer controlled four channel electrical stimulator with galvanically separated channels. Constant-current, rectangular biphasic impulses of 0.3-ms duration were delivered.
Surface EMG recordingsSurface EMG signal during electrically evoked muscle contraction and MVC was recorded with Ag-AgCl electrodes (9 mm diameter, Hellige, Freiburg, Germany). Two electrodes (2 cm inter-electrode distance) were placed over VL at 80% on the line between the anterior spina iliaca superior and the joint space in front of the anterior border of the medial ligament (Hermens and Freriks, 1997). The positions of electrodes were marked with waterproof resistance dots and these dots served as a reference for future electrode placement. The resistance of the skin was set under 5 k™¦. Direction of electrodes was aligned to the greatest M-wave at given stimulation. The SEMG signal was recorded with Myolab II (Motion Control, Inc., Salt Lake City, Utah) and sampled with frequency at 2000 Hz. For analysis of EMG signals during MVC, signals were high-pass filtered (cut-off frequency = 10 Hz, 1st order) and rectified.
M-wave measurementSingle supramaximal electrical stimulus was delivered to the femoral nerve. The current used to elicit a maximum M-wave was determined in each subject by increasing the stimulation current until no further increase in maximum M-wave amplitude were observed. This amplitude was then increased for additional 50% and used for measurements. The following parameters were obtained: peak to peak amplitude (AM-wave) and peak to peak duration (DM-wave).
Passive twitch testThree supramaximal stimuli were delivered consequently with a 1-s delay directly to the relaxed VL muscle. The current used to elicit a maximum twitch was determined in each subject by increasing the stimulation current until no further increase in torque was observed despite further increment in current. The current at maximum twitch torque was further increased by 50% to secure the supramaximal stimulation level. The torque signals from the twitch responses were smoothed (moving average; n = 5) and averaged with a trigger point at stimulus delivery. The following parameters were obtained: maximum twitch torque (TTW), contraction time (CT) and half-relaxation time (HRT).
Low and high frequency testRelaxed VL muscle was directly stimulated with two consecutive trains of impulses at 20 Hz (1s long train) and at 100 Hz (0.8s long train). Stimulation amplitude was set to three times of the motor threshold amplitude (smallest current to induce visible muscle twitch) and was kept the same for both frequencies. The mean torque during the last 100 ms of stimulation for each frequency was obtained (T20 and T100). The ratio between T20 and T100 was calculated according to the eq. 1.
Maximum voluntary knee extension testOn the command, the subject started contraction to get the maximum isometric torque approximately 2-s after the start and maintained it for 2-s. The torque signal was smoothed and analysed for maximum torque during the 1-s interval (TMVC). The SEMG parameters during the same interval were calculated: (1) average amplitude of rectified SEMG signal - AMVC(Figure 1); (2) median frequency (MFMVC) of power spectrum, which was calculated using a Fast Fourier algorithm and Hamming window function (one window with one second length) and (3) average SEMG amplitude normalised to the maximum knee torque (AMVC/TMVC).
Statistical analysisA 2x2 ANOVA for repeated measures with two factors (time and exercise) was used to test the statistical differences between SSC vs. CON exercise. The SPSS statistical package (version 15.0; SPSS Inc, Chicago, IL) was used for analysis. For all statistical analyses, a p < 0.05 was accepted as the level of significance. All the data are given as means with their standard deviations.
The SSC exercise induced a loss of HF torque from 68.0 (29.8) m before the exercise to 59.1 (26.5) Nm after the exercise (p < 0.001), while LF torque stayed virtually the same (p > 0.05) (Table 1, Figure 1). This was demonstrated in the average HLF ratio decrease by 10.9 (8.4) % (p < 0.01) (Figure 1). The CON exercise induced a loss of LF (from 37.3 (18.1) Nm to 18.7 (12.4) Nm, p < 0.001) and HF torques (from 58.2 (28.2) Nm to 38.7 (21.7) Nm, p < 0.001) simultaneously, but the amount of loss was greater for LF torque compared to HF torque (Table 1, Figure 1). This resulted in the average HLF ratio increase by 35.9 (17.5) % (p < 0.001) (Figure 1). The HLF ratio exhibited opposite behaviour after each exercise (F1,8=45.5; p < 0.001) The passive twitch contractile output of VL muscle was clearly impaired after the CON exercise, since passive twitch torque decreased on average by 51 (17.5) % (p < 0.001), although CT shortened on average by 8.7 (10.6) % (p < 0.05) (Figure 2). Meanwhile, after the CON the maximum voluntary torque was affected by only a 3.7 (9.9) % decrement (Figure 2). After the SSC exercise, passive twitch torque showed opposite behaviour as after the CON exercise, because increased twitch torque by 25.8 (14.5) % (p < 0.001) was accompanied by reduced passive twitch contraction and half relaxation time (Figure 2). The values were 9.3 (9.1) % (p < 0.05) for contraction time and 12.4 (9.4) % (p < 0.05) for relaxation time, respectively. The maximum voluntary torque was not affected after the SSC exercise (Figure 2). Peak to peak amplitude of M-waves stayed almost the same after both exercises (p > 0.05), while peak to peak duration slightly increased after the CON exercise (Figure 3). The duration increased on average by 9.2 (13.3) % (p < 0.05). Although the MVC torque was not significantly changed after the CON exercise (p > 0.05), the increment of surface EMG amplitude during MVC was substantial (by 32.9 (21.6) %, p < 0.001) (Figure 3). Consequently, change of AMVC/TMVC ratio was non significant (p > 0.05) (Figure 3). The increased amplitude of surface EMG signal during MVC was accompanied by reduced median frequency of EMG power spectrum by 11.0 (10.5) % (p < 0.05). The SSC exercise had no significant effect on myoelectrical parameters during elicited and voluntary contractions (Figure 3).
Both exercises were employed as models known to induce specific type of peripheral fatigue. As expected, the SSC exercise induced a considerable reduction in HF torque, while LF torque stayed virtually the same. The CON exercise resulted in reduced LF and HF torque, but the amount of reduction was greater in LF than HF torque. The HLF ratio exhibited specific and opposite behaviour after each exercise, noting that the SSC exercise resulted in HF peripheral fatigue and the CON exercise in LF peripheral fatigue, which was also observed in other studies (Jereb and Strojnik, 2001; 2003; Strojnik and Komi, 1998; 2000). This enabled further analysis of measured surface EMG parameters during evoked and voluntary contraction regarding specificities to different types of voluntary induced peripheral fatigue. HF fatigue induced with electrical stimulation is closely linked to alteration of muscle membrane excitability with no involvement of contractile metabolic changes (Bigland-Ritchie et al., 1979; Darques and Jammes, 1997; Darques et al., 2003; Metzger and Fitts, 1986). Indeed, after maximal SSC exercise, the electrically evoked muscle torque production capability was not impaired as showed by increased twitch torque, shorter contraction and half relaxation time (Figure 2). The SSC exercise-dependent contractile potentiation might occur due to an increase of Ca2+ sensitivity or an increase in Ca2+ release (MacIntosh and Rassier, 2002). Twitch potentiation was also accompanied with maintained LF torque (Table 1, Figure 1). A shorter passive twitch duration and consequently smaller twitch fusion was probably the main reason that potentiation during low stimulation frequencies (T20) was not observed. Although the SSC exercise clearly potentiated contractile function of VL muscle, HF torque was severely depressed. Similar results were obtained by Strojnik and Komi, 1998 after maximal SSC sledge jumps as well. Such results implied on propagation failure over the sarcolemma due to HF stimulation, which have already been seen in electrically elicited HF fatigue (Bigland-Ritchie et al., 1979; Darques and Jammes, 1997; Darques et al., 2003; Metzger and Fitts, 1986). According to that and in an agreement with mentioned studies, the SSC was expected to reduce peak to peak amplitude of maximal M-wave. However, the data of our study implied that membrane excitability was not impaired (Figure 3), since peak to peak amplitude and duration of M-wave did not change significantly. The main reason for HF torque reduction was probably the alterations in the action potential propagation distal to the sarcolemma (Balog and Fitts, 1996). Therefore it was speculated that HF torque reduction after the SSC was caused by alteration in action potential propagation at t-tubules. Since perturbations in action potential propagation at t-tubules could not be monitored with surface EMG signal, peak to peak amplitude of M-wave did not change. Interestingly, maximum voluntary torque production was also preserved (Figure 2) after the SSC, which is also in agreement with other studies (Skof and Strojnik, 2006a; 2006b; Strojnik and Komi, 1998; 2000). Preserved isometric torque was not in conflict with speculation about the alteration of action potential at t-tubules, because isometric MVC requires rather low or moderate motor unit firing frequencies around 42 Hz (Pucci, et al., 2006) or even lower (Bellemare et al., 1983), what is inside the capacity of sarcolemma and t-tubules to transmit action potentials (Sejersted and Hallèn, 1987). Since no changes in amplitude of surface EMG signal (Figure 3) and its normalisation to maximal voluntary torque were observed it is possible to conclude that after the SSC exercise voluntary muscle activation capability was preserved. The CON exercise induced different and opposite behaviour in contractile characteristics of VL muscle than the SSC exercise since passive twitch (Figure 2), LF and HF torques were impaired (Table 1, Figure 1). Passive twitch torque reduction was accompanied with twitch contraction time reduction (Figure 2) implying impaired Ca2+ transient (Balnave and Allen, 1995; Hill et al., 2001; Leppik et al., 2004) and/or reduced myofibril Ca2+ sensitivity (Cooke et al., 1988) after the CON exercise. Although contractile perturbations after CON exercise were substantial, alteration in M-wave amplitudes were not present. This has been seen also after electrically induced LF fatigue (Badier et al., 1999; Sandercock et al., 1985). There was only minor prolongation of peak to peak duration of the M-wave (Figure 3) implying slower sarcolemma AP propagation (Broman et al., 1985), which might be the consequence of lactate production in the CON exercise as observed by Jereb and Strojnik (2003) after the same protocol. Interestingly, similar response in LF and HF torques has been obtained after submaximally intensive SSC exercise as well (Skof and Strojnik, 2006a; 2006b; Strojnik and Komi, 2000) which were both related to substantial increase of blood lactate concentration. Therefore it seems that when exercise induces significant metabolic response low-frequency fatigue can be expected. Lower intracellular pH after CON exercise probably decreased muscle fibre conducting velocity, which has been seen as prolongation of peak to peak duration of the M-wave. Such view is not in agreement with interpretation of Pasquet et al., 2000 regarding high frequency fatigue after concentric contractions. Additionally, it is possible that part of HF torque reduction was not due to high-frequency fatigue but to failure of the muscle contractile apparatus resulting from metabolic response after the CON exercise. This would strengthen the view that LF fatigue was dominant after the CON exercise. As electrically evoked mechanical parameters clearly showed different peripheral origin of voluntary induced HF and LF fatigue, this could not be seen in the M-waves (Figure 3). Interestingly, isometric maximal voluntary torque production showed completely different behaviour than electrically evoked contractions. Although voluntary maximal torque production was not altered after both exercise, EMG amplitude and median frequency of the EMG power spectrum during MVC showed different behaviour. Increased amplitude of surface EMG signal was accompanied with decreased median frequency of power spectrum, but only after the CON exercise (Figure 3). Greater metabolic changes after the CON exercise could cause slower sarcolemma action potential propagation (Broman et al., 1985), resulting in changed shape of the action potentials (Basmajin and DeLuca 1985). This could be the main reason for increased amplitude of the surface EMG signal and decreased median frequency of power spectrum after the CON exercise. It has been suggested that fatigue after voluntary contractions is most probably caused by peripheral alterations (Beelen et al., 1995). Therefore, the central mechanisms were very likely not significantly involved into fatigue after CON exercise in the present study as the changes in voluntary SEMG were in line with behaviour of the M-waves.
The SSC exercise induced high-frequency fatigue which was not reflected in any SEMG change during M-wave and MVC. On the other hand, the CON exercise induced dominantly low-frequency fatigue where only SEMG during MVC changed. It is concluded that muscle fibre membrane excitability was not changed due to low- and high-frequency fatigue and that the changes after CON exercise reflected mostly metabolic changes. The changes in muscle compound action potential after voluntary induced HF fatigue did not follow the changes seen after electrically elicited HF fatigue.
ACKNOWLEDGEMENTS |
This work was supported by Slovenian Ministry of High Education, Science and Technology Grants L5-6570-0587-04 and L5-5330-0587 and Grant from Slovenian Sport Foundation. The experiments comply with the current laws of our country. |
|
AUTHOR BIOGRAPHY |
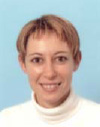 |
Katja Tomazin |
Employment: Faculty of Sport, University of Ljubljana, Slovenia. |
Degree: PhD |
Research interests: Neuromuscular fatigue, resistance training. |
E-mail: katja.tomazin@fsp.uni-lj.si |
|
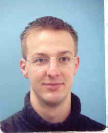 |
Nejc Sarabon |
Employment: Faculty of Sport, University of Ljubljana, Slovenia. |
Degree: PhD |
Research interests: Motor control, motor behaviour, motor learning. |
E-mail: nejc.sarabon@fsp.uni-lj.si |
|
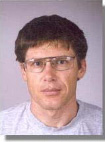 |
Vojko Strojnik |
Employment: Faculty of Sport, University of Ljubljana, Slovenia. |
Degree: MD |
Research interests: Resistance training, motor control, muscle fatigue. |
E-mail: vstr@fsp.uni-lj.si |
|
|
|
REFERENCES |
 Babcock M.A., Pegelow D.F., Taha B.H., Dempsey J.A. (1998) High frequency diaphragmatic fatigue detected with paired stimuli in humans. Medicine and Science in Sports and Exercise 30, 506-511.
|
 Badier M., Guillot C., Danger C., Tagliarini F., Jammes Y. (1999) M-wave changes after high- and low-frequency electrically induced fatigue in different muscles. Muscle & Nerve 22, 488-496.
|
 Balnave C.D., Allen D.G. (1995) Intracellular calcium and force in single mouse muscle fibres following repeated contractions with stretch. The Journal of Physiology 488, 25-36.
|
 Balog E.M., Fitts R.H. (1996) Effects of fatiguing stimulation on intracellular Na+ and K+ in frog skeletal muscle. Journal of Applied Physiology 81, 679-685.
|
 Basmajian J.V., DeLuca C.J (1985) Muscle alive. Baltimore. Wiliams & Wilkins.
|
 Beelen A., Sargeant A.J., Jones D.A., de Ruiter C.J. (1995) Fatigue and recovery of voluntary and electrically elicited dynamic force in humans. The Journal of Physiology 484, 227-235.
|
 Bellemare F., Woods J.J., Johansson R., Bigland-Ritchie B. (1983) Motor-unit discharge rates in maximal voluntary contractions of three human muscles. Journal of Neurophysiology 50, 1380-1392.
|
 Bigland-Ritchie B., Jones D.A., Woods J.J. (1979) Excitation frequency and muscle fatigue: electrical responses during human voluntary and stimulated contractions. Experimental Neurology 64, 414-427.
|
 Broman H., Billoto G., DeLuca C.J. (1985) Myoelectric signal conduction velocity and spectral parameters: influence of force and time. Journal of Applied Physiology 58, 1428-1437.
|
 Cooke R., Franks K., Luciani G.B., Pate E. (1988) The inhibition of rabbit skeletal muscle contraction by hydrogen ions and phosphate. The Journal of Physiology 395, 77-97.
|
 Darques J.L., Bendahan D., Roussel M., Giannesini B., Tagliarini F., Le Fur Y., Cozzone P.J., Jammes Y. (2003) Combined in situ analysis of metabolic and myoelectrical changes associated with electrically induced fatigue. Journal of Applied Physiology 95, 1476-84.
|
 Darques J.L., Jammes Y. (1997) Fatigue induced changes in group IV muscle afferent activity: differences between high- and low- frequency electrically-induced changes. Brain Research 750, 147-157.
|
 Finni T., Ikegawa S., Lepola V., Komi P.V. (2003) Comparison of force-velocity relationships of vastus lateralis muscle in isokinetic and in stretch-shortening cycle exercises. Acta Physiologica Scandinavica 177, 483-491.
|
 Hennings K., Kamavuako E.N., Farina D. (2007) The recruitment order of electrically activated motor neurons investigated with a novel collision technique. Clinical Neurophysiology 118, 283-291.
|
 Hermens H.J., Freriks B. (1997) The state of the art on sensors and sensorplacement procedures for surface electromyography: A proposal for sensor placement procedures. Enschede, Netherlands. Roessingh Research and Development.
|
 Hill C. A., Tompson M. W., Ruell P. A., Thom J. M., White M. J. (2001) Sarcoplasmic reticulum function and muscle contractile character following fatiguing exercise in humans. The Journal of Physiology 531, 871-8.
|
 Jereb B. (1995) Neuromuscular fatigue at maximal activity of different duration. Ljubljana. University of Ljublana.
|
 Jereb B. (1998) Neuromuscular fatigue at maximal activity of different duration. Ljubljana. University of Ljublana.
|
 Jereb B., Strojnik V. (2001) High frequency fatigue appearing after maximal hopping. Kinesiology 2, 159-167.
|
 Jereb B., Strojnik V. (2003) Neuromuscular fatigue after short maximum cycling exercise. Kinesiology 2, 135-142.
|
 Jones D.A, Porter J (1981) Human muscle fatigue: Physiological mechanisms. Muscle fatigue due to changes beyond the neuromuscular junction. and Whelan, R. London. Pitman Medical.
|
 Jones D.A. (1996) High- and low- frequency fatigue revisited. Acta Physiologica Scandinavica 156, 265-270.
|
 Leppik J.A., Aughey R.J., Medved I., Fairweather I., Carey M.F., McKenna M.J. (2004) Prolonged exercise to fatigue in humans impairs skeletal muscle Na+-K+-ATPase activity, sarcoplasmic reticulum Ca2+ release, and Ca2+ uptake. Journal of Applied Physiology 97, 1414-1423.
|
 Luttmann A., Jäger M., Witscher K., Vorgerd M., Tegenthoff M. (1998) SENIAM, Proceedings of the third general SENIAM workshop. Fatigue induced EMG changes during low-level isometric contractions. Germany. Achen.
|
 MacIntosh B.R., Rassier D.E. (2002) What is fatigue?. Canadian Journal of Applied Physiology 27, 42-55.
|
 Metzger J.M., Fitts R.H. (1986) Fatigue from high- and low frequency muscle stimulation: role of sarcolemma action potential. Experimental Neurology 93, 320-333.
|
 Pasquet B., Carpentier A., Duchateau J., Hainaut K. (2000) Muscle fatigue during concentric and eccentric contractions. Muscle & Nerve 23, 1727-1735.
|
 Pucci A.R., Griffin L., Cafarelli E. (2006) Maximal motor unit firing rates during isometric resistance training in men. Experimental Physiology 91, 171-178.
|
 Sandercock T.G., Faulkner J.A., Walbers J., Abbrech P. (1985) Single motor unit and fibre action potentials during fatigue. Journal of Applied Physiology 58, 1073-1079.
|
 Sejersted O.M., Hallén J. (1987) Na, K Homeostasis, of Skeletal Muscle during Activation. Medicine and Sport Science 26, 1-11.
|
 Skof B., Strojnik V. (2006a) Neuromuscular fatigue and recovery dynamics following prolonged continous run at anaerobic threshold. British Journal of Sports Medicine 40, 219-222.
|
 Skof B., Strojnik V. (2006b) Neuro-muscular fatigue and recovery dynamics following anaerobic interval workload. International Journal of Sports Medicine 27, 220-225.
|
 Stokes M.J., Edwards R.H., Cooper R.G. (1989) Effect of low frequency fatigue on human muscle strength and fatigability during subsequent stimulated activity. European Journal of Applied Physiology and Occupational Physiology 59, 278-283.
|
 Strojnik V., Komi P.V. (1998) Neuromuscular fatigue after maximal stretch-shortening cycle exercise. Journal of Applied Physiology 84, 344-350.
|
 Strojnik V., Komi P.V. (2000) Fatigue after submaximal intensive stretch-shortening cycle exercise. Medicine and Science in Sports and Exercise 32, 1314-1319.
|
 Tomazin K., Dolenec A., Strojnik V. (2008) High-frequency fatigue after alpine slalom skiing. European Journal of Applied Physiology , -.
|
|
|
|
|
|
|