|
|
|
ABSTRACT |
The aim of the present study was to evaluate the efficacy of intermittent hypoxic training (IHT) with 95 % of lactate threshold workload (WRLT) on aerobic capacity and endurance performance in well-trained cyclists. Twenty male elite cyclists, randomly divided into a hypoxia (H) group (n=10; age 22 ± 2.7years; VO2max 67.8 ± 2.5 ml·kg-1·min-1; body height (BH) 1.78 ± 0.05 m; body mass (BM) 66.7 ± 5.4kg; fat free mass (FFM) 59.3 ± 5.1kg; fat content (FAT%) 11.3 ± 2.1%), and a control (C) group (n = 10; age 23.5 ± 3. 5years; VO2max 67.7 ± 2.0 ml·kg-1·min-1; BH 1.79 ± 3.2 m; BM 69.2 ± 5.5 kg; FFM 63.6 ± 4.8 kg; FAT% 7.9 ± 1.94 %) took part in the research project. The training program used during the experiment was the same for the both groups. For three weeks, the subjects in H group performed 3 training sessions per week in normobaric hypoxia environment (IHT - O2 = 15. 2%). During the elemental core of the IHT session, the intensity was set at 95% WRLT for 30-min in 1st microcycle, 35-min in 2nd microcycle and 40-min in 3rd microcycle. The same training procedure was provided in C group, yet the intensity of the main sessions were set at 100% WRLT in the normoxia environment. The results indicate a significant (p < 0.05) increase in VO2max,VO2LT, WRmax, WRLT and change in lactate concentration (∆LA) during incremental test in H group. Also a significant (p < 0.05) decrease in time of the time trial was seen, associated with a significant increase (p < 0.05) in average generated power (Pavg) and average speed (Vavg) during the time trial. The intermittent hypoxic training (IHT) applied in this research did not significantly affect the hematological variables considered: number of erythrocytes (RBC), hemoglobin concentration (HGB) and haematocrit value (HCT). Significant blood value increases (p < 0.05) were only observed in MCV in H group. This data suggests that intermittent hypoxic training at lactate threshold intensity and medium duration (30-40min) is an effective training means for improving aerobic capacity and endurance performance at sea level. |
Key words:
Intermittent hypoxic training (IHT), hypoxia, aerobic capacity, endurance performance, cycling
|
Key
Points
- The efficacy of the intermittent hypoxic training is mostly dependent on volume and intensity of exercise in the hypoxic environment.
- The observed results suggests that intermittent hypoxic training at lactate threshold intensity and medium duration (30-40min) is an effective training means for improving aerobic capacity and endurance performance at sea level.
|
The concept of altitude or hypoxic training for sea-level sport performance improvement has been known for nearly 40 years. During this time, several strategies of such training regimens have been proposed, like live high - train high (LH-TH) or live high - train low (LH-TL). Another method of altitude training that has received recent attention in sport science, as well as in sport training, is the live low - train high procedure (LL-TH). In this model of altitude training athletes live under normoxic conditions and train in a natural, hypobaric or simulated normobaric hypoxic environment. Normobaric hypoxia can be simulated via nitrogen dilution, oxygen filtration or inspiration of hypoxic gas. Athletes typically use this equipment for simulated normobaric hypoxic environment at rest (intermittent hypoxic exposure; IHE) or in conjunction with physical training, referred to as intermittent hypoxic training (IHT). Theoretically, the stress of hypoxic exposure, in addition to training stress, will compound the training adaptations experienced with normal endurance training and will lead to greater improvements in performance (Wolski et al., 1996). These systematic reductions in PO2 during the training process may trigger various biochemical and structural changes in skeletal muscle that favor oxidative processes (Geiser et al. , 2001; Melissa et al., 1997; Zoll et al., 2006; Terrados et al., 1990). One of the hypothesis states that the hypoxic exposition during training (IHT), similarly to the IHE, may stimulate serum erythropoietin synthesis (sEPO), which allows for an increase in erythrocyte count and improved oxygen supply to the working muscles. (Knaupp et al., 1992; Powell et al., 2000). Unfortunately, numerous researches did not support this hypothesis (Katayama et al., 2004; Rodriguez et al., 2004; Roels et al., 2005). However, in the newest study, Hamlin et al., 2010 reported an increase in hemoglobin concentration and haematocrit value after IHT. In addition, some authors suggest that IHT may also improve anaerobic exercise performance (Bonnetti et al., 2006; Hendriksen and Meeuwsen, 2003), possibly via increases in muscle buffering capacity (Gore et al., 2001) and increased glycolytic enzyme activity (Katayama et al., 2004). In one of the recent studies (Hamlin 2010), authors reported an improvement in mean power during the 30-s Wingate test after IHT. Current research results on the effectiveness of IHT for the enhancement of sea-level performance are ambiguous. Only a few studies (Green et al., 1999; Hamlin et al., 2010; Melissa et al., 1997; Terrados et al., 1990; Zoll et al., 2006) reported enhanced endurance performance at sea-level following IHT, although a number of research projects have failed to demonstrate improvement in sea-level performance after IHT (Morton and Cable, 2005; Roels et al., 2005; Truijens et al., 2003). These conflicting reports on the efficacy of IHT in the literature may be due to methodological differences, including the type, volume and intensity of exercise in the hypoxic environment, as well as the intensity of the hypoxic stimulus and the sports level of research subjects. The current research in this area generally has focused on training, where subjects preformed prolonged exercise with low (Hamlin et al., 2010; Hendriksen and Meeuwsen, 2003) or high intensity interval training, with low volume (PPO), in a hypoxic environment (Morton and Cable, 2005; Roels et al., 2005). There is also very limited data about the efficacy of IHT on aerobic capacity and sea-level performance, where well-trained subjects trained at lactate threshold intensities in a hypoxic environment, even though this IHT protocol seems efficient for endurance performance improvement (Dufour et al., 2006; Zoll et al., 2006). On the other hand, using high intensity interval protocols (Morton and Cable, 2005; Roels et al., 2005) did not observe any significant changes in endurance performance and hematological variables. Finally, there are only few studies in competitive athletes where all training sessions were (under hypoxic and normoxic conditions) controlled and registered. Therefore, the main objective of this work was to evaluate the efficacy of intermittent hypoxic training (IHT) with 95% of lactate threshold workload on aerobic capacity and endurance performance in well-trained cyclists.
SubjectsTwenty male elite cyclists, with at least 6 years of national and international experience took part in the research project. All of the tested athletes possessed current medical examinations, stating no contradictions to perform exhaustive exercise in a hypoxic environment. All subjects were randomly divided into a hypoxia (H) group (n = 10; age 22 ± 2.7 years; VO2max 67.8 ± 2.5 ml·kg-1·min-1; body height (BH) 1.78 ± 0.05 m; body mass (BM) 66.7 ± 5.4 kg; fat free mass (FFM) 59.3 ± 5.0 kg; fat content (FAT%) 11.3 ± 2.1%), which trained three times per week in a normobaric hypoxia environment, and a control (C) group, which exercised under normoxic conditions (n = 10; age 23.5 ± 3.5 years; VO2max 67.7 ± 2. 0 ml·kg-1·min-1; BH 1.79 ± 0.03 m; BM 69.2 ± 5.5 kg; FFM 63.6 ± 4.8 kg; FAT% 7.9 ± 1.9 %). The research project was approved by the Ethics Committee for Scientific Research at the Academy of Physical Education in Katowice, Poland.
Experimental designThe research was conducted during the second phase of the preparatory period (March 2010). During this training phase, a considerable amount of training volume is dedicated for lactate threshold improvement. The experiment had two series of testing, separated by four microcycles of training (25 days). The first series of testing was conducted at the beginning of the experiment to determine initial values of analyzed variables. Three microcycles (3 weeks) with progressive training loads were then applied, followed by a fourth recovery microcycle in which the training load was significantly reduced. After the recovery microcycle, the last series of testing was preformed. The testing procedure in the second series of testing was exactly the same as in first. Because of the high training intensity during the experiment, all subjects were on a high carbohydrate diet (Carbohydrates - 60%, Protein -15%, Fats - 25%). The average daily intake of carbohydrates was set at 6-7g/kg of body mass.
Experimental testingEach series of testing included two days of investigations. On the first day, before breakfast, resting blood samples were drawn from the antecubical vein to determine hematological variables (hemoglobin concentration (HGB), haematocrit value (HCT), number of erythrocytes (RBC)). Body mass and body composition were then evaluated by electrical impedance (Inbody 720, Biospace Co., Japan). Three hours after a light breakfast, a progressive ergocycle test was administered to determine maximal oxygen uptake and lactate threshold. Capillary blood samples were drawn at rest, after each workload and during the 3rd, 6th, 9th, and 12th min of recovery for the evaluation of lactate concentration (LA). Also, capillary rest and post-exercise blood samples were used to determine acid-base equilibrium and oxygen saturation of hemoglobin. After resting blood samples were drawn for biochemical evaluations, a progressive test was applied to determine maximal oxygen uptake and lactate threshold (LT). The LT was determined by the D-max method (Cheng et al., 1992), because in one of our previous studies (Czuba et al., 2009), we observed that the workload associated with lactate threshold, as determined by the D-max method during a progressive test, was not significantly different from that determined for the maximal lactate steady state (MLSS), for both male and female cyclists. The progressive test (T3x40) was performed on an ergocycle Excalibur Sport (Lode BV, Netherlands), beginning with a workload of 80W, which was increased by 40W every 3 minutes until volitional exhaustion. Starting at a higher load and increasing it by a greater margin (50W) would not allow for a precise determination of the lactate threshold, which was one of the major objectives of this paper. The criteria of reaching VO2max included a plateau in values of VO2 or a gradual decrease in peak VO2 during the maximal workload. During the progressive test, the following variables were constantly registered: heart rate (HR) (S810i, Polar Electro, Finland), minute ventilation (VE), oxygen uptake (VO2) and expired carbon dioxide (CO2) (MetaLyzer 3B-R2, Cortex, Germany). During this test all cyclists were instructed to remain seated. After 24h of rest, all subjects preformed a simulation 30km time trial in a hilly terrain. While performing the time trial the subjects were occasionally allowed to take a standing position to improve their riding comfort. Every test was performed on a individual bike of each cyclist, which was connected to a Virtual Reality cycle trainer (Fortius, Tacx). Resting and post-exercise blood samples were drawn from the antecubical vein to determine creatine kinase activity (CK) and values of HGB, HCT and RBC. Also, capillary blood samples were drawn at rest, after each 10 km of the time trial (10, 20, 30km) to determine lactate concentration (LA), acid- base equilibrium and oxygen saturation of hemoglobin. During the time trial, the following variables were constantly registered: heart rate, speed, cadence and power (PowerTap Pro+, CycleOps). Before every time trial, the cycletrainer and power meter were calibrated for each bike. During the test, subjects could ingest only ad libitum water. The same experimental procedure was applied during the second series of testing.
Training programThe training program used during the experiment was the same for both groups, but with individual training zones for every cyclist (Table 1). For three microcycles (three weeks), the subjects in group H preformed three training sessions per week in a normobaric hypoxic environment (O2=15.2%; simulation of 2,500-2,600m). Each training sessions in the hypoxic environment consisted of a 15-min warm-up, followed by 30-40 min of elemental core training and a cool-down of 15-min. The warm-up resistance was set at 60% of the lactate threshold workload (WRLT) for each cyclist for 5-min; then increased by 10% of WRLT every 5-min (60%-5min, 70%-5min, 80%- 5min). During the core element of the session, the intensity was set at 95% WRLT for 30-min in the first microcycle, 35-min in second microcycle and 40-min in third microcycle. After a 15-min cool- down in the hypoxic environment, where resistance was set at 55% WRLT, cyclists in group H preformed a 2h ride at 60-75% WRLT under normoxia conditions. In the present study the intensity related to the lactate threshold (%WRLT) during every hypoxic and normoxic training session in both groups was registered in watts and controlled by power meters (PowerTap Pro+, CycleOps). The same training procedure was provided for group C, but the intensity during the main part of the training session was set at 100% WRLT in comparison to the normoxia environment. These training sessions were also conducted in the laboratory, with the hypoxic generator set at a concentration of oxygen equal to 21%. The normobaric hypoxia environment was simulated by a HYP-123 Hypoxic Generator (Hypoxico). During the hypoxic training sessions the HYP-123 generator was connected to the Altitude Trainer (Hypoxico), where airflow was 126 l/min (7600 l/hr). None of the subjects in group H had problems with higher ventilation rates, because minute ventilation in this group at lactate threshold workload oscillated between 85 -100 l/min. Also, the workload applied during the core of IHT sessions were 5% lower than WRLT. The subjects in H group during hypoxia sessions trained on their own bicycles, fixed on an electromagnetic trainer (Fortius, Tacx); therefore, the laboratory training was very similar to a natural cycling training. The cyclists drank ad libitum electrolyte drinks through a straw. During every hypoxic session in group H, FIO2 (In-Line O2 Port with Handi O2 Monitor, Maxtec), heart rate (S810i, Polar Electro) and blood oxygen saturation SpO2 were registered (Pulsox-3, Minolta). In the hypoxic (H) group, values of SpO2 during the core element of IHT session varied from 80 to 85%, whereas the control (C) group’s SpO2 remained between 95-94%. Besides registering the intensity of the training process during the first and last hypoxic training session, capillary blood samples were drawn to determine LA, acid-base balance and saturation of hemoglobin. The blood samples were taken at rest, after warm-up and during the core element of the protocol, every 10 minutes (10, 20, 30, 40 minutes). During the second microcycle, additional blood samples were drawn at the end of the session (35-min). It must be underlined, that the lactate concentration was significantly higher in group H - lactate paradox, but its concentration did not raise more than 1 mmol·l-1 in the last 20 minutes of the final the trainings session. Also, at the beginning of each microcyle, and after one day off, resting blood samples were drawn from the antecubical vein to determine changes in hematological variables (HGB, HCT, RBC).
Statistical analysisThe obtained data were analyzed statistically with the use of Statistica 9.0 (StatSoft). The results were presented as arithmetic means and standard deviations (SD). To determine the influence of training in a normobaric hypoxia environment on aerobic capacity and cycling performance at sea level, the two-way ANOVA (group & training) with repeated measures was applied. When significant differences in F ratio were found, the post hoc Tukey’s test was used. To determine significant differences in values of lactate concentration between the first and last IHT session, the t-test for paired samples was applied. The level of statistical significance was set at p < 0.05.
The average values of the variables during the progressive ergocycle test and time trial (TT), are presented in Tables 2 and 3">3. The two- way analysis of variance showed a statistically significant effect of the two main factors (group & training) on registered variables during progressive ergocycle test, such as: maximal oxygen uptake (VO2max), oxygen uptake at the lactate threshold workload (VO2LT), maximal workload (WRmax), lactate threshold workload (WRLT), maximal minute ventilation (VEmax) and delta (∆) values of lactate concentration (∆LA) (Table 2). Additionally, a significant effect of the two main factors during the time trial (TT) occurred in the final result expressed in time (T), average power (Pavg), average heart rate (HRavg), delta values of creatine kinase activity (∆CK) and lactate concentration (Table 3). The intermittent hypoxic training (IHT) applied in this research did not significantly effect the analyzed hematological variables of RBC, HGB, HCT and red blood cell distribution width (RDW). Significance within the two main factors occurred only in MCV values (Table 4). The training program used in this research did not affect body mass and body composition significantly (Table 5).
Post hoc analysisThe statistical analysis indicated that the IHT caused a significant (p < 0.05) increase in maximal workload (WRmax) during the incremental test (T3x40) by 6.6% in H group, as well as an 8,3% increase in the workload measured at the lactate threshold. During the experiment, absolute values of maximal oxygen uptake (VO2max) increased significantly (p < 0.05) by 4% in H group. Similar changes were observed in values of oxygen uptake at lactate threshold (VO2LT) in this group, which increased significantly (p < 0.05) by 9.1%. Also, values of maximal minute ventilation (VEmax) and ∆LA during T3x40 in H group were significantly (p < 0.05) higher in comparison to baseline levels. Training in C group did not caused significant changes in any of the above variables. Additionally, a significant (p < 0.05) decrease by 2.6% in time of TT was observed after 3 weeks of IHT, which was accompanied by a significant (p<0.05) increase in Pavg (5.6%) (Table 3). The analysis of the generated power during TT in both groups demonstrate that values of average power generated between 10 and 20 km (Pavg 10-20km), as well as between 20 and 30 km (Pavg 20-30km), were significantly (p < 0.05) increased by 4.7% and 6.9%, respectively, after IHT (Figure 1). The changes in the average power generated at the first 10km (Pavg 0-10km) were non-significant in both groups, but there was tendency towards increased values. A significant (p < 0.05) increase in Pavg and non-significant changes in body mass (BM) in H group caused significant (p < 0.05) increases in values of average speed (Vavg) during TT, by 2.8%. Also a significant (p < 0.05) decrease in average heart rate (HRavg) during TT occurred after IHT. Non-significant changes in HRmax during T3x40 in H group could have been caused by a significant (p < 0.05) increase in maximal workload (WRmax) in this group. The IHT caused a significant decrease (p < 0. 05) in the delta (∆) values of CK by 23.3% during TT. Additionally, there were marked decreases in delta (∆) values of lactate concentration after 10 km (∆LA0-10km) and 20 km (∆LA0-20km), but only changes in ∆LA0-20km in H group were significantly (p<0.05) decreased (23.7%). However, inversely proportional changes occurred in the delta (∆) values of lactate concentration after 30km (∆LA0-30km). The ∆LA0-30km significantly increased (p < 0.05) by 27.4% after IHT. However, t- test results showed that lactate concentration during the first and last IHT session significantly decreased in group H. The values of chosen hematological variables, such as red blood cells (RBC), hemoglobin concentration (HGB), hematocrit value (HCT) and red blood cell distribution width (RDW), were not affected by IHT (Table 4). However, there was a tendency for a slight increase in the resting values of these variables after IHT. The only significant increase (p < 0.05) observed was in values of mean cell volume (MCV) in H group (Table 4).
The aim of present study was to determine whether IHT with lactate threshold workload intensity would enhance sea- level aerobic capacity and endurance performance in well-trained cyclists. The unique aspect of this research project included highly trained athletes and a well designed intensive training program. The most important finding from the present study is that the 3 week training program, associated with 3 IHT sessions per week (180min per week, where 90 - 120min per week corresponded to a workload equal to 95% of the lactate threshold), significantly improved aerobic capacity (VO2max by 4%) and endurance performance (time in TT by 2.6%) in well-trained cyclists at sea-level. However, only a few well-designed and well-controlled studies on trained subjects have reported increments in hemoglobin concentration or/and hematocrit value (Bonetti et al., 2006; Gore et al., 2006 Hamlin 2010), while other studies do not show significant changes in serum erythropoietin and erythrocyte count following IHE/IHT (Katayama et al., 2004; Marshall et al., 2008; Rodriguez et al., 2004; Roels et al., 2005). Efficiency of the oxygen transport system is most often evaluated by maximal oxygen uptake (VO2max). Theoretically, intermittent hypoxic training (IHT) may increase aerobic capacity and endurance performance at sea-level by several adaptive changes. However, research findings on IHT as an effective protocol for enhancing aerobic capacity and sport performance at sea-level are inconclusive. Few well-controlled studies support this theory (Dufour et al., 2006; Zoll et al., 2006). Dufour et al., 2006 observed a significant improvement in VO2max, and in running speed at the second ventilatory threshold (VT2), by 5% and 4%, respectively, after 6 weeks of intermittent hypoxic training, without changes in blood O2-carrying capacity. Moreover, the time in an all- out running test increased only in the experimental group, without significant modifications in VO2 kinetics. The subjects in the control and experimental groups continued their usual running training program with two additional sessions with intensity at the second ventilatory threshold. Athletes in the experimental group preformed these sessions in a hypoxic environment. In another study, Roels et al., 2005 also observed a significant improvement in values of VO2max after high intensive interval (IHT) sessions, but there were non-significant differences in mean power output during a 10- min cycle time trial and hematological variables after IHT. However, the IHT intensive interval sessions, as well as hypoxic exposure, were only twice a week (~115min per week). The interval training consisted of 6-8 reps of 2min duration at 100% peak power output (PPO) during the first 4 wk; then progressively increased to 5 reps of 5-6 min at 90% of PPO, followed by 4 reps of 8 min at 90% of PPO in the last week of training. It is difficult to pinpoint the exact causes of such training effects, yet they could have been affected by the short duration, as well as low overall volume, of interval work for well-trained athletes. In most available data, the overall volume of the hypoxic exposure compared to our study was significantly lower However, many studies do not confirm the ergogenic effects of IHT. The results of Roels et al., 2005 investigations are in accordance with those of Truijens et al., 2003, who found that 5 weeks of high-intensity training in a flume improved sea-level performance in well- trained swimmers, but there was no additional effect of hypoxic training (15.3% oxygen equivalent). Similarly, in a previous study, Vallier et al., 1996 found no significant increase in VO2max and maximal power output in five elite triathletes after IHT. During the experiment these subjects modified their usual training program and replaced three sessions of bicycling exercise with three sessions on a cycle ergometer in a hypobaric chamber simulating an altitude of 4000 m. However, the authors did not include a control group, so it is not possible to compare the relative effects of this program. Hendriksen and Meeuwsen, 2003 conducted an experiment in which they examined the effect of intermittent training in a hypobaric chamber on physical exercise at sea-level. During this study, subjects in the experimental group trained for 10 days, 2 h daily on a cycle ergometer placed in a hypobaric chamber at a simulated altitude of 2,500 m. Training intensity was at 60-70% of the heart rate reserve. The results indicated a significant (p < 0.05) increase in anaerobic capacity, but not in maximal oxygen uptake VO2max. These results were confirmed by recent study of Hamlin et al., 2010. During the experiment the authors used a very similar training protocol but the fraction of inspired oxygen (FIO2) was manually adjusted to allow a similar hypoxic stimulus for each subject. The SpO2 levels were 88% on days 1-2, 84% on days 3-4 and 82% on days 5-10 (the equivalent of 3200, 4000, and 4400m altitudes, respectively). Authors observed enhanced in main anaerobic power during a 30-s Wingate test, as well as found substantial increases in the hemoglobin concentration, hematocrit, and substantial reductions in serum iron and transferrin following IHT. However, there was a significant improvement in 20 km time trial. The lack of improved in aerobic capacity end endurance performance is this studies was most likely caused by hypoxia-induced low intensity efforts during the IHT workouts. In our opinion, intensity of 60-70% of the heart rate reserve is not a sufficient training stimulus, and will not lead to improvements in aerobic capacity. In our study the training load during the core element of practice sessions was significantly higher and reached on the average 250W while the HR ranged from 175 to 180 bpm. The results of our study are in accordance to those obtained by Dufour et al., 2006 and Zoll et al., 2006. We observed a significant (p < 0.05) increase in absolute and relative values of VO2max and VO2LT after the three weeks of IHT protocol. Additionally, a significant increase in WRmax and WRLT occurred, similarly to changes observed by Dufour et al., 2006. Moreover, the analysis of heart rate (HR) in our research indicated significant changes in exercising heart rates in group H. In this group, values of HRavg during the time trial were significantly lower after IHT, even though Pavg increased substantially. There was also a tendency for lower HRmax values during the incremental test, but non-significant differences in values of this variable were caused by significant increases (6.6%) in WRmax. The changes in exercise HR (decreased at a set load) may be explained by increased stroke volume, what caused an improvement in values of oxygen pulse. These adaptive changes most likely allowed for a more effective work of the cardiovascular system. An increase in maximal oxygen uptake due to hypoxia is usually associated with an increase in hematocrit value and hemoglobin concentration. In the present study, improved VO2max after IHT cannot be explained by changes in blood variables, as no significant changes in hemoglobin concentration, hematocrit value and red blood cell distribution width were observed, yet the IHT showed a tendency for increased values of this variables. Statistical analysis showed only a significant increase (p < 0.05) in values of mean corpuscular volume (MCV) in H group. Not many well designed studies reported increments in hemoglobin concentration and/or hematocrit value (Bonetti et al., 2006; Gore et al., 2006; Hamlin 2010; Hamlin and Hellemans, 2007), while other studies showed no significant changes in serum erythropoietin and erythrocyte count following IHE/IHT (Katayama et al., 2004; Marshall et al., 2008; Rodriguez et al., 2004; Roels et al., 2005). These discrepancies related to the effectiveness of IHE/IHT are most likely caused by differences in the time course of the EPO response in humans, due to hypoxia. Knaupp et al., 1992 examined the relationship between the duration of normobaric hypoxic exposure and plasma EPO levels in healthy human subjects. No increase in EPO was seen after the 5- and 60-min exposures. However, a 50% increase was seen 240 min after the initiation of the 120-min hypoxic exposure (p < 0.01). Intermittent exposure resulted in an increase of EPO by 52%, 360 min after the onset of exposure (p < 0.05). They conclude that exposing humans continuously to an inspiratory O2 fraction of 0.105 for 120 min or intermittently for 240 min provides a sufficient stimulus to increased production of EPO. Since EPO blood concentration rises significantly after 90 minutes of continues exercise, the time of the exercise protocol seems fully justified. Additionally these adaptive changes require systematic frequent training stimulus with the volume ranging form 90 to 120 minutes. These findings were confirmed by Rodriguez et al., 2000, who stated that a single hypoxic (4000-5000 m) exposure of 90 min, three times per week for three consecutive weeks, significantly (p < 0.05) increases hematocrit value (HCT), hemoglobin concentration (HGB), the number of red blood cells (RBC) and reticulocytes. These authors suggest that a continuous exposure of 90 min represents the minimal stimulus to trigger an acute secretion of erythropoietin (EPO) (hypobaric chamber at 504 - 540 hPa). However, no significant differences were found in cycling exercise time or VO2max, and no normoxic control group was included. Moreover, Dufour et al. (2006), as well as Roels et al. (2005), observed improvements in VO2max without changes in blood O2-carrying capacity. It must be underlined, that the significant increase in values of VO2max and improvement in cycling performance observed also in our study after IHT is associated with non-hematological adaptive mechanisms due to hypoxia. The results of our and several well-controlled studies indicate that the improvement in aerobic capacity and endurance performance are caused by muscular and systemic adaptations, which are either absent or found to a lesser degree after training under normoxic conditions. (Dufour et al., 2006; Zoll et al., 2006). The systematic hypoxic condition during the training process may cause more drastic changes in muscle tissues than after traditional endurance training in normoxic conditions are related increased skeletal muscle mitochondrial density, capillary-to-fiber ratio, and fiber cross- sectional area, which were demonstrated in untrained individuals (Desplanches and Hoppeler, 1993; Vogt et al., 2001). These adaptive changes are associated with an increase hypoxia inducible factor-1α (HIF-1α), which is the global regulator of oxygen homeostasis and plays a critical role in the cardiovascular and respiratory responses to hypoxia (Semenza, 2004). In another three different studies (Green et al., 1999; Melissa et al., 1997; Terrados et al., 1990) that also used the IHT protocols have demonstrated significant (p < 0.05) increases in the activities of oxidative enzymes and in capillary density associated with VO2max improvement. There have been relatively few studies that have investigated changes in monocarboxylate transporters, MCT1 and MCT4, to altitude exposure (Clark et al., 2004; Gore, 2007; Zoll et al., 2006). Zoll et al., 2006 reported a significant increase in muscle mRNA concentration of MCT1 in nine well-trained runners after IHT, compared with a control group. The authors concluded that the increase in MCT1 mRNA allowed for an improved lactate exchange and removal, which may lead to a slower decline in pH at a given running velocity, thereby allowing the athletes to run longer (Zoll et al., 2006). These applications were confirmed in our study, where a significant increase (p < 0.05) in WRLT and decrease (p < 0.05) in ∆ LA0-20km during the time trial after IHT was noted. However, while values of LA0-30km and ∆ LAmax during the incremental test were significantly higher, this was associated and accountable due to much higher workloads in group H. In addition, transport of LA and H+, as well as the ability of skeletal muscle to buffer H+, are important for pH regulation, and changes in acid-base status have been proposed as a potential mechanism for improved performance after altitude exposure (Gore, 2007). Previous studies (Mizuno et al., 1990; Saltin et al., 1995) reported that training in a hypoxia environment may increase muscle buffering capacity in well- trained athletes. Despite that our results did not show significant changes in blood pH and acid- base balance at rest, during and after the incremental exercise, as well as the time trial, there were smaller disturbances in these variables at particular loads. Also there was a significant improvement in maximal and average generated power during TT after IHT. This may suggest that the buffering capacity was increased after IHT.
In summary, the most important findings of this work include a significant increase in VO2max, VO2LT, WRmax and WRLT after three weeks of intermittent hypoxic training (IHT) with 95% of lactate threshold intensity. Additionally, the intermittent hypoxic training caused a significant increase in average power (Pavg) and average speed (Vavg) during the time trial (TT), as well as significantly reduced time of TT in group H. During the experiment, no significant changes were noticed in values of chosen hematological variables of red blood cells (RBC), hemoglobin concentration (HGB), hematocrit value (HCT) and red blood cell distribution width (RDW). No changes were registered in the acid- base balance due to IHT, but there was an improvement in performance. The results of this study and literature review, allow us to conclude that intermittent hypoxic training with high intensity (close to and above upper lactate threshold) and medium duration (30-40min) is an effective training means for improving aerobic capacity and endurance performance.
|
AUTHOR BIOGRAPHY |
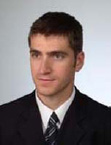 |
Milosz Czuba |
Employment: |
Degree: PhD |
Research interests: Endurance training, altitude training, sports nutrition, exercise physiology |
E-mail: m.czuba@awf.katowice.pl |
|
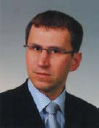 |
Zbigniew Waskiewicz |
Employment: Jerzy Kukuczka Academy of Physical Education in Katowice, Poland.Department of Team Sport Games |
Degree: PhD |
Research interests: |
E-mail: |
|
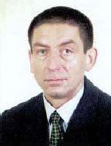 |
Adam Zajac |
Employment: Jerzy Kukuczka Academy of Physical Education in Katowice, Poland.Department of Sports Training. |
Degree: PhD |
Research interests: Strength training, sports nutrition, exercise physiology |
E-mail: a.zajac@awf.katowice.pl |
|
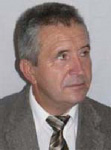 |
Stanislaw Poprzecki |
Employment: Jerzy Kukuczka Academy of Physical Education in Katowice, Poland.Department of Biochemistry |
Degree: PhD |
Research interests: Biochemical markers of overtraining, sports nutrition, hormonesand exercise. |
E-mail: s.poprzecki@awf.katowice.pl |
|
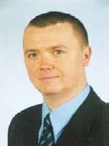 |
Jaroslaw Cholewa |
Employment: Jerzy Kukuczka Academy of Physical Education in Katowice, Poland. Department of Sports Training. |
Degree: PhD |
Research interests: Youth sports, development of motor abilities. |
E-mail: j.cholewa@awf.katowice.pl |
|
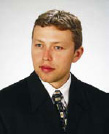 |
Robert Roczniok |
Employment: Jerzy Kukuczka Academy of Physical Education in Katowice, Poland.Department of Statistics and Methodology |
Degree: PhD |
Research interests: Artificial neural networks, multidimensional analysis, taxonomic analysis, control of sport training |
E-mail: r.roczniok@awf.katowice.pl |
|
|
|
REFERENCES |
 Bonnetti D.L., Hopkins W.G., Kilding A.E (2006) High-intensity kayak performance after adaptation to intermittent hypoxia. International Journal of Sports Physiology and Performance 1, 246-260.
|
 Cheng B., Kuipers H., Snyder A.C., Keizer H.A., Jeukendrup A., Hesselink M (1992) A new approach for the determination of ventilator and lactate thresholds. International Journal of Sport Medicine 13, 518-522.
|
 Clark S.A., Aughey R.J., Gore C.J., Hahn A.G., Townsend N.E., Kinsman T.A., Chow C.M., McKenna M.J., Hawley J.A. (2004) Effects of live high, train low hypoxic exposure on lactate metabolism in trained humans. Journal of Applied Physiology 96, 517-525.
|
 Czuba M., Zajac A., Cholewa J., Poprzecki S., Waskiewicz Z (2009) Lactate threshold (D-max method) and maximal lactate steady-state in cyclists. Journal of Human Kinetics 21, 49-56.
|
 Desplanches D., Hoppeler H (1993) Effects of training in normoxia and normobaric hypoxia on human muscle ultrastructure. Pflügers Archiv European Journal of Physiology 425, 263-267.
|
 Dufour S.P, Ponsot E., Zoll J., Doutreleau S., Lonsdorfer-Wolf E., Geny B., Lampert E., Flück M., Hoppeler H., Billat V., Mettauer B., Richard R, Lonsdorfer J (2006) Exercise training in normobaric hypoxia in endurance runners. I. Improvements in aerobic performance capacity. Journal of Applied Physiology 100, 1238-1248.
|
 Geiser J., Vogt M., Billeter R., Zuleger C., Belforti F., Hoppeler H (2001) Training high-living low: changes of aerobic performance and muscle structure with training at simulated altitude. International Journal of Sports Medicine 22, 579-585.
|
 Gore C.J., Clark S.A., Saunders P.U (2007) Nonhematological mechanisms of improved sea-level performance after hypoxic exposure. Medicine and Science in Sports and Exercise 39, 1600-1609.
|
 Gore C.J., Hahn A.G., Aughey R.J., Martin D.T., Ashenden M.J., Clark S.A., Garnham A.P., Roberts A.D., Slater G.J., McKenna M.J (2001) Live high:train low increases muscle buffer capacity and submaximal cycling efficiency. Acta Physiologica Scandinavica 173, 275-286.
|
 Gore C.J., Rodriguez F.A., Truijens M.J., Townsend N.E., Stray-Gundersen J., Levine B.D (2006) Increased serum erythropoietin but not red cell production after 4 wk of intermittent hypobaric hypoxia (4,000-5,500 m). Journal of Applied Physiology 101, 1386-1393.
|
 Green H., MacDougall J., Tarnopolsky M., Melissa N.L (1999) Downregulation of Na-K-ATPase pumps in skeletal muscle with training in normobaric hypoxia. Journal of Applied Physiology 86, 1745-1748.
|
 Hamlin M.J., Hellemans J (2007) Effect of intermittent normobaric hypoxic exposure at rest on haematological, physiological and performance parameters in multi-sport athletes. Journal of Sports Sciences 25, 431-441.
|
 Hamlin M.J., Marshall C.H., Hellemans J., Ainslie P.N., Anglem N (2010) Effect of intermittent hypoxic training on a 20 km time trial and 30 s anaerobic performance. Scandinavian Journal of Medicine and Science in Sports 20, 651-661.
|
 Hendriksen I.J.M., Meeuwsen T (2003) The effect of intermittent training in hypobaric hypoxia on sea-level exercise: a cross-over study in humans. European Journal of Applied Physiology 88, 396-403.
|
 Katayama K., Sato K., Matsuo H., Ishida K., Iwasaki K., Miyamura M (2004) Effect of intermittent hypoxia on oxygen uptake during submaximal exercise in endurance athletes. European Journal of Applied Physiology 92, 75-83.
|
 Knaupp W., Khilnani S., Sherwood J., Scharf S., Steinberg H (1992) Erythropoietin response to acute normobaric hypoxia in humans. Journal of Applied Physiology 73, 837-840.
|
 Marshall H.C., Hamlin M.J., Hellemans J., Murrell C., Beattie N., Hellemans I., Perry T.L., Burns A., Ainslie P.N (2008) Effects of intermittent hypoxia on SaO2. cerebral and muscle oxygenation during maximal exercise in athletes with exercise-induced hypoxemia. European Journal of Applied Physiology 104, 383-393.
|
 Melissa L., Macdougall J.D., Tarnopolsky M.A., Cipriano N., Green H.J (1997) Skeletal muscle adaptations to training under normobaric hypoxic versus normoxic conditions. Medicine and Science in Sports and Exercise 29, 238-243.
|
 Mizuno M., Juel C., Bro-Rasmussen T., Mygind E., Schibye B., Rasmussen B., Saltin B (1990) Limb skeletal muscle adaptation in athletes after training at altitude. Journal of Applied Physiology 68, 496-502.
|
 Morton J.P., Cable N.T (2005) The effects of intermittent hypoxic training on aerobic and anaerobic performance. Ergonomics 48, 1535-1546.
|
 Powell F.L., Garcia N (2000) Physiological effects of intermittent hypoxia. High Altitude Medicine and Biology 1, 125-136.
|
 Rodriguez F.A., Truijens M.J., Townsend N.E., Martini E.R., Stray-Gundersen J., Gore C.J., Levine B.D (2004) Effects of four weeks of intermittent hypobaric hypoxia on sea level running and swimming performance. Medicine and Science in Sports and Exercise 36, 338-.
|
 Rodriguez F.A., Ventura J.L., Casas M., Casas H., Pages T., Rama R., Ricart A., Palacios L., Viscor G (2000) Erythropoietin acute reaction and haematological adaptations to short, intermittent hypobaric hypoxia. European Journal of Applied Physiology 82, 170-177.
|
 Roels B., Millet G.P., Marcoux C.J.L., Coste O., Bentley D.J., Candau R.B (2005) Effects of hypoxic interval training on cycling performance. Medicine and Science in Sports and Exercise 37, 138-146.
|
 Saltin B., Larsen H., Terrados N., Bangsbo J., Bak T., Kim C.K., Svedenhag J., Rolf C.J (1995) Aerobic exercise capacity at sea level and at altitude in Kenyan boys, junior and senior runners compared with Scandinavian runners. Scandinavian Journal of Medicine and Science in Sports 5, 209-221.
|
 Semenza G.L (2004) O2-regulated gene expression: transcriptional control of cardiorespiratory physiology by HIF-1. Journal of Applied Physiology 96, 1173-1177.
|
 Terrados N., Jansson E., Sylven C., Kaijser L (1990) Is hypoxia a stimulus for synthesis of oxidative enzymes and myoglobin?. Journal of Applied Physiology 68, 2369-2372.
|
 Terrados N., Melichna J., Sylvén C., Jansson E., Kaijser L (1988) Effects of training at simulated altitude on performance and muscle metabolic capacity in competitive road cyclists. European Journal of Applied Physiology 57, 203-209.
|
 Truijens M.J., Toussaint H.M., Dow J., Levine B.D (2003) Effect of high-intensity hypoxic training on sea-level swimming performances. Journal of Applied Physiology 94, 733-743.
|
 Vallier J.M., Chateau P., Guezennec C.Y (1996) Effects of physical training in a hypobaric chamber on the physical performance of competitive triathletes. European Journal of Applied Physiology and Occupational Physiology 73, 471-478.
|
 Vogt M., Puntschart A., Geiser J., Zuleger C., Billeter R., Hoppeler H (2001) Molecular adaptations in human skeletal muscle to endurance training under simulated hypoxic conditions. Journal of Applied Physiology 91, 173-182.
|
 Wolski L.A., McKenzie D.C., Wenger H.A (1996) Altitude training for improvements in sea level performance: is there scientific evidence of benefit?. Sports Medicine 22, 251-263.
|
 Zoll J., Ponsot E., Dufour S., Doutreleau S., Ventura-Clapier R., Vogt M., Hoppeler H., Richard R., Fluck M (2006) Exercise training in normobaric hypoxia in endurance runners. III. Muscular adjustments of selected gene transcripts. Journal of Applied Physiology 100, 1258-1266.
|
|
|
|
|
|
|