|
|
|
ABSTRACT |
To investigate the feasibility of developing a method for detection of gene doping in power-athletes, we devised an experimental model system. Myostatin is a potent negative regulator of skeletal muscle development and growth, and myostatin-knockout mice exhibit a double-muscle phenotype. To achieve knockdown, we constructed plasmids expressing short hairpin interfering RNAs (shRNAs) against myostatin. These shRNAs were transfected into C2C12 cultured cells or injected into the tibialis anterior (TA) muscle of adult mice. By performing in vitro and in vivo experiments, we found that some shRNAs effectively reduced the expression of myostatin, and that the TA muscle showed hypertrophy of up to 27.9%. Then, using real-time PCR, we tried to detect the shRNA plasmid in the serum or muscles of mice into which it had been injected. Although we were unable to detect the plasmid in serum samples, it was detectable in the treated muscle at least four weeks after induction. We were also able to detect the plasmid in muscle in the vicinity of the TA. This gene doping model system will be useful for further studies aimed at doping control. |
Key words:
In vitro and in vivo transformation, C2C12 cultured cell, tibialis anterior, real-time PCR, shRNA
|
Key
Points
- Using a myostatin knockdown plasmid, we have succeeded in creating a model system for gene doping using mice that resulted in muscle hypertrophy greater than that reported previously.
- We confirmed that there was a limit of gene doping detection using real-time PCR, either from serum or muscle smple.
- This model experimental system can be utilized for examining indirect methods of gene doping detection such as immune responses to gene transfer or a profiling approach using DNA microarray.
|
In 1807, a cattle breed with extraordinary muscle hypertrophy was reported in the UK (Culley, 1807). Belgian Blue and Piedmontese are cattle breeds with this characteristic, and their offspring are bred as domestic animals in Europe (Dickman, 1997). In these cattle, the number of muscle fibers at birth is approximately double that of normal cattle (Bellinge et al., 2005), individual fibers are larger (Swatland and Kieffer, 1974), fast-type muscle fibers are predominant (West, 1974), and there is a low content of collagen and fatty tissues (Hanset, 1991; Hocquette et al., 1999). McPherron et al. reported that these cattle bear mutations in the myostatin gene, whose product normally works to limit skeletal muscle mass (McPherron and Lee, 1997). Therefore, it has been considered that the muscle hypertrophy in these mutants is due to “loss of myostatin function”, and this myostatin-related hypertrophy has been demonstrated in knockout mice showing the double muscle phenotype (McPherron et al., 1997). After the discovery of myostatin, a number of animal species with a double muscling phenotype attributable to a myostatin mutation were reported. One of these was the whippet, a breed of racing dog that normally has a streamlined body shape, a pointed face, and features specialized for fast runner. “Bully” whippets, on the other hand, show the double-muscle phenotype caused by mutation of the myostatin gene (Shelton and Engvall, 2007). Furthermore, loss of even one functional myostatin allele seems to confer a competitive advantage on racing whippets, providing the first definitive evidence that loss of myostatin function can enhance athletic performance (Mosher et al., 2007). A similar human case has also been confirmed. In 2004, the New England Journal of Medicine reported a so-called “super baby” with skeletal muscle enlargement and enhanced physical abilities (Schuelke et al., 2004). At six days of age, ultrasonography showed that the baby had almost double the normal muscle mass, and at 4.5 years of age the child was able to hold two 3-kg dumbbells in horizontal suspension with the arms extended. Physical examination revealed no particular problems except for muscle hypertrophy, hypoglycemia and increased levels of testosterone and insulin-like growth factor I. The mother had been a former professional athlete, and some members of the family pedigree were reported to have an unusually strong physique, suggesting that the condition was genetically inherited. Gene therapy is now becoming an important technology for medical treatment, and further progress is anticipated with further improvements in biotechnology (Gabhann et al., 2010; Parker et al., 1999; Quezada et al., 2004). It is now applicable for the treatment of many diseases including forms of progressive myopathy such as muscular dystrophy (Romero et al., 2004). On the other hand, in the field of sports, anti-doping authorities fear that “gene doping” will become a means by which athletes can improve their performance (Schneider and Friedmann, 2006). Gene doping is a threat, because not only is it illegal and a health hazard, but also virtually undetectable by currently available methods (Baoutina et al., 2007). Athletes will naturally be tempted to gain muscle mass and improve their performance by manipulation of the myostatin gene or its product. In fact, injection of neutralizing monoclonal antibodies directed against myostatin into wild-type mice does increase muscle mass and strength, suggesting that myostatin can still play an important role in regulation of muscle growth in adult animals (Bogdanovich et al., 2002; Whittemore et al., 2003). With regard to gene manipulation, methods that are applicable to adults are clearly important, as it would be ethically unacceptable to create a myostatin-knockout human baby or a human mutant with a Cre-LoxP-driven myostatin knockdown system. By analogy with gene therapy, various techniques for gene doping can be envisaged. For athletes other than bodybuilders, the idea of gene doping using a virus vector seems unsuitable because it would induce systemic muscle hypertrophy. Ideally, athletes need to enlarge specific muscles that are used predominantly for their own type of sport. At present, therefore, the best approach would appear to be the use of RNA interference (RNAi) (Magee et al., 2006). RNAi is a recently developed technique for gene manipulation in adults, and here we describe a gene doping model using RNAi that induces muscle hypertrophy. No previous report has described a method for detection of gene doping (Baoutina et al., 2008), despite the urgent need to develop such a method for effective use in sport. Here we describe a trial for detection of gene doping using RNAi, and refer to the limitations of currently available detection methods.
Plasmid used for myostatin knockdownWe analyzed the mouse myostatin mRNA sequence (NCBI Reference Sequence: NM_010834.2) to find potential siRNA targets using siDirect version 2.0 software (http://design.RNAi.jp/) with the help of the developer’s suggestions. Three candidates were identified as knockdown sequences for myostatin: K1 (5'- GGAATCCGATCTCTGAAACTTG-3' nucleotide positions 668-689), K2 (5'-GGCACTGGTATTTGGCAGA- 3' nucleotide positions 701-719) and K3 (5'-GATGACGATTATCACGCTA- 3' nucleotide positions 428-446). These siRNAs were cloned into the piGENE™hU6 Puro plasmid vector (Clontech, USA) as short hairpin DNA sequences in accordance with the manufacturer’s instructions. This vector contains the human U6 promoter that drives RNA polymerase III, and transcribes shRNA (short hairpin RNA) from the downstream insert DNA. Synthesized oligonucleotides, sense and antisense strands used for making short hairpin DNAs were as follows: K1 sense; 5'-CACCGGAATCCGATCTTTGGAATTTGTGTGCTG TCCAAGTTTCAGAGATCGGATTCCTTTTTT- 3' K1 antisense; 5'-GCATAAAAAAGGAATCCGATCTCTGAAACTTG GACAGCACACAAATTCCAAAGATCGGATTCC- 3' K2 sense; 5'-CACCAGGCATTGGTGTTTGGTAGAGGTGTGCT GTCCCTCTGCCAAATACCAGTGCCTTTTTTT- 3' K2 antisense; 5'-GCATAAAAAAAGGCACTGGTATTTGGCAGAGG GACAGCACACCTCTACCAAACACCAATGCCT- 3' K3 sense; 5'-CACCGATAACGACTACCACGCTAACGTGTGCT GTCCGTTAGCGTGATAATCGTAATCTTTTT- 3' K3 antisense; 5'-GCATAAAAAGATGACGATTATCACGCTAACGG ACAGCACACGTTAGCGTGGTAGTCGTTATC- 3' Annealed dsDNAs were ligated to the BspMI site of piGENETMhU6 Puro (Clontech, USA), and used for transformation of DH5. In the cell, this vector produces an shRNA with a hairpin loop structure that can be processed by Dicer to yield siRNA. We also constructed a positive control plasmid for transformation using electroporation. Transformation efficiency was monitored using the CAG promoter-driven pCAGGS plasmid with a lacZ gene insert at the EcoRI site. Each plasmid was prepared with an EndFree Plasmid Mega Kit (Qiagen, USA) and stocked in a deep freezer until use.
Knockdown of myostatin expression in cultured myocytesC2C12 myoblasts derived from mouse skeletal muscle were cultured with DMEM (GIBCO, USA) containing 10% FBS and 1% antibiotics in a 5% CO2 atmosphere at 37 °C. We used LipofectamineTM2000 (Invitrogen, USA) for gene transfection into myocytes. Upon lipofection, we used DMEM without antibiotics for 24 hours before induction. The cell density for lipofection was adjusted to 90% - 95%. Four micrograms of plasmid (U6, K1, K2 and K3) and 10 µl of Lipofectamine were separately dissolved in 250 µl of OPTI-MEM (Invitrogen, USA), and mixed gently for 20 min at room temperature. Cultured myocytes were washed with PBS and transferred to 2 ml of OPTI-MEM. After a 20-minute incubation, 500 µl of the plasmid/ Lipofectamine mixture was poured into a culture dish containing the cells, and incubation was carried out at 37 °C for 6 hours. We then exchanged the culture medium with the original DMEM containing 10% FBS and 1% antibiotics. Selection of infected cells was done in DMEM containing 5 µg/ml puromycin for 24 hours after lipofection had been conducted for 24 hours. We subcultured the selected cells for 48 hours in 12-well plates (for RNA preparation) and 6-well plates (for protein preparation).
Experimental approval and animal careAnimal experiments were carried out in a humane manner after receiving approval from the Institutional Animal Experiment Committee of the University of Tsukuba, and in accordance with the Regulations for Animal Experiments at the University and the Fundamental Guidelines for Proper Conduct of Animal Experiments and Related Activities in Academic Research Institutions under the jurisdiction of the Ministry of Education, Culture, Sports, Science and Technology of Japan.
Knockdown of myostatin expression in mouse muscleWe used female ICR mice 7 weeks of age that had been housed in an animal facility under a 12-12 h light-dark regime at 23 ± 2°C and 55 ± 5% humidity, and fed a rodent chow and water ad libitum. Diluted pentobarbital sodium salt (5 mg/ml; Nakarai Tesque, Japan) was injected intraperitoneally (85 mg/kg body weight) for anesthetization. Cnemial hair was shaved off, and the skin in front of the tibialis anterior (TA) muscle was exposed. Twenty-five microliters of plasmid solution (U6, K2 or K3; 4 µg/µl each) was injected into the TA using a Rhodos 29 G syringe (BD, USA). We divided the injection to allow diffusion of the plasmid into the whole TA muscle. An Electro Square Porator ECM 830 (BTX Harvard Apparatus, USA) with a 2-Needle Array™ electrode was used for electroporation. Two electrodes separated by 5 mm were inserted into the TA transversely, and four electrical stimulations (1 Hz, 20 ms) of 100 V were given. We performed a further four electrical stimulations using a reverse current field. At 1-6 weeks after electroporation transformation, the TA muscle was dissected from each mouse after sacrifice by cervical dislocation. We measured the wet weight of the muscle, and placed it on an ice-cooled aluminum block. For histological study, the muscle sample was embedded in OCT compound and stored at -30 °C. For mRNA and protein analyses, it was cut into small pieces with a scalpel, mixed into a uniform mass, and stored at -80 °C after immediate freezing with liquid nitrogen.
Quantification of mRNA expression levelTotal RNA was extracted from cultured cells or excised muscle using TRIzol reagent (Invitrogen, USA). cDNA was reverse-transcribed with oligo (dT) primer using ReverTraAce (Toyobo, Japan). Semi-quantitative real-time PCR was performed on an ABI PRISM 7500 (Applied Biosystems, USA) using SYBR Green Master mix (Applied Biosystems, USA). The PCR comprised 50 cycles of 95 °C for 15 seconds and, 60 °C for 60 seconds, and another step of 95 °C for 15 seconds, 60 °C for 60 seconds and 95 °C for 15 seconds was added to allow derivation of the dissociation curve. The level of expression in the sample was estimated as a relative value by reference to a standard curve, and normalized against HPRT (hypoxanthine phosphoribosyltransferase). The primer sets used for analysis were as follows. myostatin; forward 5'-CTGAGACTCATCAAACCCATGAAA- 3' reverse 5'-TGCCTGGGCTCATGTCAAG- 3' HPRT; forward 5'-GGTTTTGCTCAGTGGAATAAACATG- 3' reverse 5'-AAAAGGAACTGTTGACAACGATTTACT- 3'
Quantification of introduced vector using serum or muscle samplesGenomic and plasmid DNA was extracted from serum using a QIAamp Circulating Nucleic Acid Kit (QIAGEN, Germany), and from skeletal muscle using phenol-chloroform. Plasmid DNA was quantified by real-time PCR (TAKARA Thermal Cycler Dice® Real Time System, Japan) using SYBR® Premix Ex Taq™ (TAKARA, Japan). The PCR comprised 40 cycles of 95 °C for 5 seconds, and 65 °C for 30 seconds, and another step of 95 °C for 15 seconds, 65 °C for 30 seconds and 95 °C for 15 seconds was added to allow derivation of the dissociation curve. The primer set used was as follows. Beta-lactamase (penicillin resistance) gene in plasmid; forward 5'- CGGATGGCATGACAGTAAGAGA -3' reverse 5'- ACGATCAAGGCGAGTTACATGA -3'
Quantification of protein contentCultured cells or frozen muscle samples were collected and homogenized in lysis buffer (150 mM NaCl, 1% NP-40, 0.5% sodium deoxycholate, 0.1% SDS, 5 mM benzamidine, 1 mM EDTA, 5 mM N-ethylmaleimide, 25 mM β- glycerophosphate, 1 mM sodium orthovanadate, 50 mM NaF, 1 mM PMSF, 1/100 protease inhibitor cocktail, 50 mM HEPES pH 7.9), and then centrifuged. The precipitate was discarded, and the supernatant was solubilized in SDS sample buffer (2% SDS, 25% glycerol, 5% 2-mercaptoethanol, 0.01% bromophenol blue, 62.5 mM Tris-HCl pH 6.8) and heat-treated at 37 °C for one hour. The concentration of all the samples was adjusted to 0.5 µg/µl. After SDS-PAGE through 10% polyacrylamide gel, samples were transferred to PVDF membrane at 300 mA for 14 hours. In advance of immunoblotting, the membranes were blocked with 5% skim milk in TBS-T (0.05% Tween 20 in Tris- buffered saline) at room temperature for one hour. The membranes were incubated with the following three primary antibodies at 4 °C for 24 hours. Subsequently, they were incubated with a secondary antibody (alkaline phosphatase-conjugated anti-mouse or anti-rabbit IgG; Biosource International, USA) at room temperature for one hour. Target bands were detected with a BCIP-NBT solution kit (Nakarai Tesque, Japan) and analyzed with NIH Image 1.63 (National Institutes of Health, USA) software.
Histochemical analysis for estimation of transformation efficiencyUsing pCAGGS-LacZ, we estimated the efficiency of transformation by electroporation. Whole muscle was rinsed in PBS for 10 minutes and dipped into staining solution (1mg/ml X-gal, 5mM K3 [Fe(CN)6] (III), 5 mM K4 [Fe(CN)6] (II), 2 mM MgCl2 in PBS) without previous fixation and incubated at 37 °C for 24 hours. The muscle was then washed in PBS three times for 10 minutes each time, and photographed. Using a cryostat (Microme Cryo-Star HM560; Microme, Germany), we also prepared tissue sections 10 µm thick from the muscle embedded in OCT compound. Each section was placed on a glass slide, washed three times with PBS, and dipped in the above staining solution at 37 °C for 24 hours. The sections were washed in PBS three times and overlaid with a cover glass. From the micrographs that were captured into a computer, all the fibers and X-gal-stained fibers were counted, and the transformation ratio was calculated. For HE staining, we used Carrazzi’s Hematoxylin solution (Wako, Japan) and Eosin Y solution (Wako, Japan). Fiber CSA was determined by using a computer tablet to trace the outlines of immunostained muscle fibers using NIH image 1.63. The length on the micrograph was calibrated by a scale on a slide micrometer.
Statistical analysesAll experimental data are expressed as mean ± standard error. We examined the homoscedasticity for all groups. If significant probability was >0.05, we performed one-way layout analysis of variance, followed by Tukey post-hoc test. If significant probability was <0.05, we conducted the Kruskal-Wallis test, followed by the Wilcoxon rank sum test, and examined the inter-group significances. Time-dependent change in TA muscle weight was examined by two-way ANOVA. Outcome measures between two groups were compared by the unpaired t-test. The SPSS 10.0J statistical analysis software package was used.
Effects of myostatin knockdown in cultured C2C12 myocytesFour kinds of plasmid, U6, K1, K2 and K3, were constructed, introduced into C2C12 myocytes, and the transformed cells were selected using puromycin. Consequently, K1 plasmid-treated cells were found to have low drug tolerance, and therefore we did not use them in further experiments. We next determined the levels of myostatin expression in K2 and K3 transformants by real-time PCR. After transformation by lipofection, we performed puromycin selection for 24 hours, and then returned the transformants to the original culture medium for 48 hours. The expression level of myostatin mRNA was assayed by real-time RT-PCR using the expression of the HPRT housekeeping gene as a standard. We were able to confirm a significant decrease of myostatin mRNA expression in K2 and K3 as compared with the U6 control (Figure 1a). The level of myostatin protein was assayed by Western blotting, but this revealed no significant differences among the K2, K3 and U6 transformants (Figure 1b,c).
Effects of myostatin knockdown in mouse tibialis anterior muscleIn vivo effects of myostatin knockdown were assayed in mouse TA muscle. First, we examined the efficiency of transformation using pCAGGS-LacZ. Two weeks after transformation by electroporation, the TA muscle was dissected out and stained with X-gal. Microscopic observation of the sections showed that the proportion of positively stained fibers, i.e. those that had been transformed, was approximately 37%. Using the same protocol as that employed for pCAGGS-LacZ, we transformed the TA muscle with the U6, K2 and K3 plasmids, and then 2 weeks later transformation, we sampled the transformed TA muscle and measured the change in the expression level of myostatin mRNA and protein in the muscle. Myostatin mRNA was assayed by real-time PCR using a housekeeping gene (HPRT) as an internal standard, and myostatin protein expression was analyzed by Western blotting. Compared with the U6 transformant, myostatin mRNAs were decreased by 90.9% (K2) and 88.4% (K3), while myostatin protein was decreased by 27.0% (K2) and 34.2% (K3), respectively (Figure 2). Since the levels of expression of myostatin mRNA and protein were significantly decreased by K2 and K3 transformation, muscle mass and fiber size were analyzed two weeks after transformation. As expected, muscle wet weight and fiber size were increased in the K2 (12.6% and 53.7%) and K3 (27.9% and 40.3%) transformants, as compared with U6 (Figure 3). These results confirmed that muscle mass had been increased by RNAi against the myostatin gene.
Detection of knockdown vector in transformed miceUsing real-time PCR, we tried to detect the presence ofgene doping using both treated TA muscle tissue and circulating blood. We chose primer sets for the beta-lactamase (ampicillin resistance) gene, because almost all plasmids used for gene manipulation have this gene, and hence this primer set would be applicable for gene doping detection in the future. We sampled TA muscle and blood weekly until six week after K3 plasmid introduction. The time-dependent change in muscle wet weight is shown in Figure 4, along with corresponding TA muscle controls. A tendency for myostatin-knockdown muscle to be heavier was evident in the first and second weeks (p < 0.1), and the difference between myostatin-knockdown and control muscle was significant in the third week (p < 0.05). Thereafter, the knockdown effect decreased. Real-time PCR allowed us to detect the introduced plasmid from blood samples, but it was impossible at any time point during the six-week period (Figure 5a). On the other hand, we were able to detect the introduced plasmid in TA muscle until the fourth week, but after the fifth week it became undetectable (Figure 5b). We also tried to detect the introduced plasmid inmuscles neighboring the TA, such as the extensor digitorum longus (EDL), gastrocnemius (GAS), plantaris (PLA) and soleus (SOL) three days after shRNA vector introduction. Although we failed to detect the plasmid in the GAS, PLA and SOL, we were able to amplify the gene from the EDL, which is located adjacent to the TA (Figure 6).
Since myostatin was first identified as a negative regulator of muscle growth, many studies have demonstrated that decreasing the level of myostatin or inhibiting its function can drastically increase muscle mass. Using rodent models, several strategies have been employed so far to manipulate muscle mass by modulation of myostatin signaling or biological activity employing rodent models, several strategies have been employed so far to manipulate muscle mass by modulation of myostatin signaling or biological activity employing anti-myostatin antibodies (Bogdanovich et al., 2002; Whittemore et al., 2003) myostatin receptor competitors (Lee et al., 2005), myostatin propeptide (Wolfman et al., 2003), truncated myostatin protein (Siriett et al., 2007), follistatin (Lee, 2007; Lee and McPherron, 2001), histone deacetylase inhibitor (Iezzi et al., 2004; Minetti et al., 2006) and other approaches (Fedoruk and Rupert, 2008). These strategies may have therapeutic application for patients with myopathy or muscle atrophy, whereas if they were to be applied in healthy individuals, they would likely stimulate muscle growth and enhance performance in athletes (Whittemore et al., 2003). From an anti-doping viewpoint, clinical sports scientists have been trying to develop techniques for detecting these non-DNA/RNA substances using isoelectric focusing, immunoassay, and mass spectrometry (Azzazy et al., 2005; Kohler et al., 2010; 2011). As a consequence of legitimate gene therapy trials, gene doping has emerged as a new approach to doping in sport. Advances in gene transfer technology in humans, originating from existing gene therapy technology, may soon be utilized for enhancement for physical performance in athletes. The World Anti-Doping Agency (WADA) claims that gene doping threatens the integrity of sport, undermines the principles of fair play in sport, and involves major health risks to athletes who undertake it. In athletes other than bodybuilders (who aim to increase their muscle mass systemically), who use specific skeletal muscles for different sports, gene doping would obviously be a tempting option for targeted muscle enlargement and improvement of performance. For this purpose, systemically effective virus vectors, particularly retroviruses, are not suitable because their genetic information is incorporated into the host genome by integrase, thus leaving conclusive evidence of gene doping. Therefore, transient plasmid vectors that work in a limited part of the body and are broken down by tissue nuclease are most likely to be used for this purpose. In the present study, we adopted a shRNA plasmid against myostatin mRNA and carried out both in vitro and in vivo knockdown experiments. We confirmed in vitro that RNAi induced a significant decrease of myostatin mRNA, whereas the level of myostatin protein was not changed. This may have been due to the time lag required for RNAi to exert its effect. Seventy-two hours might not be enough for decay of preexisting myostatin protein. Therefore for the in vivo experiment, we analyzed TA muscle two weeks after introduction of shRNA. At that time point, both myostain mRNA and protein were decreased significantly. Consequently, the wet weight and myofiber cross-sectional area of the targeted muscle were increased by local gene doping. Magee et al. succeeded in knockdown of myostatin expression in 2-3-month-old male Fisher rats using almost the same procedure as ours (Magee et al., 2006). They injected a pSilencer 2.1 U6-neo based knockdown plasmid into TA, and after 2 weeks, myostatin mRNA and protein were decreased by 26.7% and 48.3%, respectively. In our knockdown experiments with 7-week-old female ICR mice, myostatin mRNA was decreased by 90.9% (K2) and 88.4% (K3), while myostatin protein was decreased by 27.0% (K2) and 34.2% (K3), respectively. Magee et al. reported that, as a result of myostatin knockdown, TA muscle wet weight was increased by 9.8% and the cross-sectional area of its myofibers was increased by 34.2%. In our experiment, on the other hand, TA wet weight was increased by 12.6% (K2) and 27.9% (K3), while the cross-sectional area of its myofibers was increased by 53.7% (K2) and 40.3% (K3), respectively. We speculate that the differences between Magee’s results and ours were attributable to the difference in the experimental animals and/or vector system employed, although both studies used RNAi with a shRNA system, and the siRNA sequence of K3 (19mer) overlapped perfectly with that used by Magee et al (21mer). Magee et al used randomer shRNA as negative control for knock-down, but we used mock vector instead and this may induce discrepancy between two experiments. Since many muscle hypertrophy mutants have been developed in mice, it is thought that the murine myostatin knockdown system will be useful for future studies of the interaction with myostatin. As for myostatin knockdown/knockout effects on fiber type of skeletal muscle, the absence of myostatin reported to lead to an overall faster and more glycolytic muscle phenotype (Girgenrath et al., 2005; Magee et al., 2006). We checked the change of MHCs mRNA in the transformed TA muscle, but levels of MHCI, MHCIIa and MHCIIb were not significantly changed as compared with those in the control muscle (data not shown). We speculated two weeks after transformation is too late for detecting changes at mRNA level. We would like to further investigate the effects of myostatin knockdown on fiber type change in skeletal muscle by MHC electrophoresis or immunohistochemical methods in the future study. We then tried to detect gene doping using the experimental system we had developed. Since expression-manipulated products, either enhanced or reduced, are likely indistinguishable from their endogenous counterparts, they cannot be detectable. Therefore, we tried to detect the introduced shRNA vector by real-time PCR. As the template for amplification, any plasmid sequence of non-mammal origin was thought to be useful, so we chose the sequence of an ampicillin resistance gene (β-lactamase), which is generally included in common plasmid vectors, and therefore would be quite useful for detection of plasmid vectors once this method has become established. At first, we thought it is unlikely to detect introduced plasmid from urine sample, so we tried to detect the introduced shRNA vector in samples of serum, which is one of the most easily accessible body fluids. From weeks 1 to 6 after shRNA introduction, we failed to detect the vector at any time point by real-time PCR. In a previous study using plasmid DNA, after intramuscular administration to mice, plasmid DNA (encoding the gene for the malaria circumsporozoite protein for malaria vaccination) was detected in blood after 1 h and 2 days, but was absent on day 7 (Parker et al., 1999), whereas in another study, plasma from all tested animals was negative for plasmid DNA (encoding the antisense gene targeting epidermal growth factor receptor for tumor growth inhibition) at 48 h and time points thereafter (Thomas et al., 2003). Plasmid DNA in plasma seems to have a very short half-life due to rapid degradation by plasma nucleases, clearance by the liver via phagocytic cells, or retention in capillary beds (Dash et al., 1999; Oh et al., 2001). As the next step for detection of gene doping, we looked for the presence of plasmid DNA in targeted muscle samples, and succeeded in amplifying the plasmid from the TA until 4 weeks after introduction. Using a plasmid encoding the human developmentally regulated endothelial locus-1 protein, Quezada et al. reported that plasmid DNA persisted at the intramuscular injection site in mice for 28 days, but was barely detectable in distal tissues by 24 h and was essentially cleared by 28 days (Quezada et al., 2004). Four weeks seems to be the maximum retention period after gene doping using plasmid DNA. Moreover, we checked all of the muscles of the lower leg, and succeeded in detecting plasmid DNA in the extensor digitorum longus, which is located close to the TA. An introduced plasmid may move to neighboring muscle by electroporation, but it would not cross the fascia cruris (i.e., the septum intermusculare anterius cruris and the membrana interossea cruris). Although muscle biopsy for doping tests is not currently applicable to athletes, our result raises the possibility that gene doping might be detectable from muscles other than the target tissue. Otherwise, we should challenge other methods for detecting gene doping from blood or urine samples, utilizing transcriptome or proteome. Sharp proposed the notion of athleticogenomics which involves the integration of selected physiologic performance parameters with genome dataset information, together with athleticoproteomics which deals with proteomics regarding trainability (Sharp, 2010). We think competitors' passports, containing record of main exercise physiologic protein or transcript parameters, will be necessary for viewing notable alterations. Technology for gene silencing is rapidly advancing. Takeshita et al have noticed that atelocollagen improves the delivery of chemically unmodified siRNA to metastatic tumors in vivo (Takeshita et al., 2005), and Kinouchi et al have reported that local and systemic application of siRNA against myostatin coupled with atelocollagen is able to markedly stimulate muscle growth in vivo within a few weeks (Kinouchi et al., 2008). Nuclease activity against siRNA can be prevented (Takei et al., 2004), and cellular uptake of siRNAs is increased by atelocollagen (Minakuchi et al., 2004). Adachi et al have found that siRNA complexed with a synthetic collagen, poly-(Pro-Hyp-Gly), is resistant to nucleases and is efficiently transferred into cells, allowing long-term gene silencing in comparison with atelocollagen in vivo (Adachi et al., 2010). They speculated that the synthetic collagen they employed formed a protective outer layer that shielded the siRNA core. These approaches facilitated increased cellular uptake, nuclease resistance and prolonged release of siRNAs, although isoelectric focusing, immunoassay, and mass spectrometry might be able to detect these artificially modified collagens. Acosta et al microinjected dsRNA against myostatin into zebrafish at the early developmental (1-2 cell) stage, and found that this increased body mass through hyperplasia and hypertrophy (Acosta et al., 2005). Although this method was effective for piscine transformation, it is unlikely to be applicable to mammalian cells that have high nuclease activity (Dash et al., 1999). Moreover, gene manipulation of early-stage human embryo in order to enhance muscle proliferation for athletic purpose is neither practical nor ethical. Recently, Kang et al have successfully performed exon skipping of myostatin in C2C12 cultured myocytes using an antisense oligonucleotide and achieved knock down of myostatin expression (Kang et al., 2011). They used phosphorodiamidate morpholino oligomers conjugated with octa-guanidine dendrimer to make them resistant to enzymatic degradation. However, when applied in vivo to TA muscle, the muscle mass was not significantly increased, and systemic intravascular treatment resulted in a significant increase of muscle mass only in the soleus. Antisense-induced exon skipping approaches for muscular dystrophy have already been used clinically to partly correct the mutated dystrophin and convert the severe Duchenne muscular dystrophy phenotype into a milder Becker dystrophy phenotype (Kinali et al., 2009; van Deutekom et al., 2007). Low genotoxicity and long-term sustainability make this method applicable to humans, but in the case of myostatin exon skipping, further investigation of delivery routes, dosing regimens and antisense oligonucleotides sequences will be necessary. The prospects for gene doping still remain essentially theoretical at present (Wells, 2008), it will necessary to monitor these approaches carefully as they have the potential to form the vanguard of future gene-doping technology.
Using a myostatin knockdown plasmid of a type most likely to be used for gene doping in power-athletes, we have succeeded in creating a mouse model system for gene doping that resulted in muscle hypertrophy greater than that reported previously. Furthermore, we confirmed that there was a limit of gene doping detection using real-time PCR, although the sensitivity was comparable to the best reported so far. We intend to utilize this model experimental system for examining indirect methods of gene doping detection such as immune responses to gene transfer or a profiling approach using DNA microarray.
ACKNOWLEDGEMENTS |
This work was supported in part by grant-in-Aid for Scientific Research (A) from the Ministry of Education, Culture, Sports, Science and Technology of Japan (#21240062). |
|
AUTHOR BIOGRAPHY |
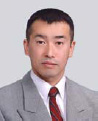 |
Tohru Takemasa |
Employment: Associate Professor at Doctoral Program in Physical Education, Health and Sport Sciences, Graduate School of Comprehensive Human Sciences, University of Tsukuba, Japan |
Degree: |
Research interests: Exercise physiology of skeletal muscle at molecular level. Resistance training induced muscle hypertrophy, fast-to-slow fiber type change through endurance training, disuse atrophy. |
E-mail: takemasa@taiiku.tsukuba.ac.jp |
|
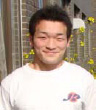 |
Naohisa Yakushiji |
Employment: Graduate from Master’s Program in Health and Sport Sciences, Graduate School of Comprehensive Human Sciences, University of Tsukuba, Japan |
Degree: MSc |
Research interests: Resistance training induced muscle hypertrophy and gene doping. |
E-mail: yakushijinaohisa@yahoo.co.jp |
|
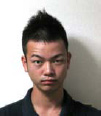 |
Dale Manjiro Kikuchi |
Employment: Student at Master’s Program in Biological Sciences, Graduate School of Life and Environmental Sciences, University of Tsukuba, Japan |
Degree: BSc |
Research interests: Resistance training induced muscle hypertrophy and gene doping. |
E-mail: dale-kikuchi@hotmail.co.jp |
|
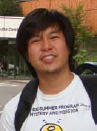 |
Custer Deocaris |
Employment: Research fellow at Doctoral Program in Physical Education, Health and Sport Sciences, Graduate School of Comprehensive Human Sciences, University of Tsukuba, Japan |
Degree: PhD |
Research interests: Molecular biology and bioinformatics of skeletal muscle and brain in exercise training. |
E-mail: cdeocaris@gmail.com |
|
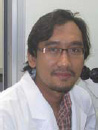 |
Widodo |
Employment: Lecturer at Biology department, Faculty of Sciences, Brawijaya University, Indonesia |
Degree: PhD |
Research interests: Cellular aging and cancer process, drug discovery based on specific target and natural product, nutrigenomic and medicinal bioinformatics. |
E-mail: widodo@ub.ac.id |
|
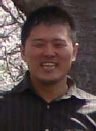 |
Masanao Machida |
Employment: Research Center for Stem Cell Engineering, National Institute of Advanced Industrial Science and Technology, Higashi, Tsukuba, Japan |
Degree: PhD |
Research interests: Judo, Exercise physiology, Epigenetics |
E-mail: masanao.m@gmail.com |
|
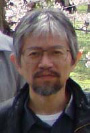 |
Hidenori Kiyosawa |
Employment: Department of Environmental Medicine, Kochi Medical School, Kochi University, Oko-cho Kohasu, Nankoku-shi, Kochi, Japan |
Degree: PhD |
Research interests: Genomics and transcriptomics of endogenous antisense RNAs/non-coding RNAs and their relationships to epigenetic regulation. |
E-mail: akiyosaw@mail.ecc.u-tokyo.ac.jp |
|
|
|
REFERENCES |
 Acosta J., Carpio Y., Borroto I., Gonzalez O., Estrada M.P. (2005) Myostatin gene silenced by RNAi show a zebrafish giant phenotype. Journal of Biotechnology 119, 324-331.
|
 Adachi T., Kawakami E., Ishimaru N., Ochiya T., Hayashi Y., Ohuchi H., Tanihara M., Tanaka E., Noji S. (2010) Delivery of small interfering RNA with a synthetic collagen poly(Pro-Hyp-Gly) for gene silencing in vitro and in vivo. Development Growth and Differentiation 52, 693-699.
|
 Azzazy H. M., Mansour M.M., Christenson R.H. (2005) Doping in the recombinant era: strategies and counterstrategies. Clinical Biochemistry 38, 959-965.
|
 Baoutina A., Alexander I. E., Rasko J.E., Emslie K.R. (2007) Potential use of gene transfer in athletic performance enhancement. Molecular Therapy 15, 1751-1766.
|
 Baoutina A., Alexander I. E., Rasko J.E., Emslie K.R. (2008) Developing strategies for detection of gene doping. Journal of Gene Medicine 10, 3-20.
|
 Bellinge R. H., Liberles D. A., Iaschi S. P., O’Brien P.A., Tay G.K. (2005) Myostatin and its implications on animal breeding: a review. Animal Genetics 36, 1-6.
|
 Bogdanovich S., Krag T. O., Barton E. R., Morris L. D., Whittemore L. A., Ahima R.S., Khurana T.S. (2002) Functional improvement of dystrophic muscle by myostatin blockade. Nature 420, 418-421.
|
 Culley G. (1807) . Observations on livestock. London. G. Woodfall.
|
 Dash P. R., Read M. L., Barrett L. B., Wolfert M.A., Seymour L.W. (1999) Factors affecting blood clearance and in vivo distribution of polyelectrolyte complexes for gene delivery. Gene Therapy 6, 643-650.
|
 Dickman S. (1997) Gene mutation provides more meat on the hoof. Science 277, 1922-1923.
|
 Fedoruk M.N., Rupert J.L. (2008) Myostatin inhibition: a potential performance enhancement strategy?. Scandinavian Journal of Medicine and Science in Sports 18, 123-131.
|
 Gabhann F. M., Annex B.H., Popel A.S. (2010) Gene therapy from the perspective of systems biology. Current Opinion in Molecular Therapeutics 12, 570-577.
|
 Girgenrath S., Song K., Whittemore L.A. (2005) Loss of myostatin expression alters fiber-type distribution and expression of myosin heavy chain isoforms in slow- and fast-type skeletal muscle. Muscle & Nerve 31, 34-40.
|
 Hanset R. (1991) Breeding for disease resistance in farm animals. , -.
|
 Hocquette J. F., Bas P., Bauchart D., Vermorel M., Geay Y. (1999) Fat partitioning and biochemical characteristics of fatty tissues in relation to plasma metabolites and hormones in normal and double-muscled young growing bulls. Comparative Biochemistry and Physiology. Part A, Molecular and Integrative Physiology 122, 127-138.
|
 Iezzi S., Di Padova M., Serra C., Caretti G., Simone C., Maklan E., Minetti G., Zhao P., Hoffman E. P., Puri P.L., Sartorelli V. (2004) Deacetylase inhibitors increase muscle cell size by promoting myoblast recruitment and fusion through induction of follistatin. Developmental Cell 6, 673-684.
|
 Kang J. K., Malerba A., Popplewell L., Foster K., Dickson G. (2011) Antisense-induced myostatin exon skipping leads to muscle hypertrophy in mice following octa-guanidine morpholino oligomer treatment. Molecular Therapy 19, 159-164.
|
 Kinali M., Arechavala-Gomeza V., Feng L., Cirak S., Hunt D., Adkin C., Guglieri M., Ashton E., Abbs S., Nihoyannopoulos P., Garralda M. E., Rutherford M., McCulley C., Popplewell L., Graham I. R., Dickson G., Wood M. J., Wells D. J., Wilton S. D., Kole R., Straub V., Bushby K., Sewry C., Morgan J.E., Muntoni F. (2009) Local restoration of dystrophin expression with the morpholino oligomer AVI-4658 in Duchenne muscular dystrophy: a single-blind, placebo-controlled, dose-escalation, proof-of-concept study. Lancet Neurology 8, 918-928.
|
 Kinouchi N., Ohsawa Y., Ishimaru N., Ohuchi H., Sunada Y., Hayashi Y., Tanimoto Y., Moriyama K., Noji S. (2008) Atelocollagen-mediated local and systemic applications of myostatin-targeting siRNA increase skeletal muscle mass. Gene Therapy 15, 1126-1130.
|
 Kohler M., Schanzer W., Thevis M. (2011) RNA interference for performance enhancement and detection in doping control. Drug Testing and Analysis 3, 661-667.
|
 Kohler M., Thomas A., Walpurgis K., Schanzer W., Thevis M. (2010) Mass spectrometric detection of siRNA in plasma samples for doping control purposes. Analytical and Bioanalytical Chemistry 398, 1305-1312.
|
 Lee S.J. (2007) Quadrupling muscle mass in mice by targeting TGF-beta signaling pathways. PLoS One 2, e789-.
|
 Magee T. R., Artaza J. N., Ferrini M. G., Vernet D., Zuniga F. I., Cantini L., Reisz-Porszasz S., Rajfer J., Gonzalez-Cadavid N.F. (2006) Myostatin short interfering hairpin RNA gene transfer increases skeletal muscle mass. Journal of Gene Medicine 8, 1171-1181.
|
 McPherron A. C., Lawler A.M., Lee S.J. (1997) Regulation of skeletal muscle mass in mice by a new TGF-beta superfamily member. Nature 387, 83-90.
|
 Minakuchi Y., Takeshita F., Kosaka N., Sasaki H., Yamamoto Y., Kouno M., Honma K., Nagahara S., Hanai K., Sano A., Kato T., Terada M., Ochiya T. (2004) Atelocollagen-mediated synthetic small interfering RNA delivery for effective gene silencing in vitro and in vivo. Nucleic Acids Research 32, e109-.
|
 Minetti G. C., Colussi C., Adami R., Serra C., Mozzetta C., Parente V., Fortuni S., Straino S., Sampaolesi M., Di Padova M., Illi B., Gallinari P., Steinkuhler C., Capogrossi M. C., Sartorelli V., Bottinelli R., Gaetano C., Puri P.L. (2006) Functional and morphological recovery of dystrophic muscles in mice treated with deacetylase inhibitors. Nature Medicine 12, 1147-1150.
|
 Mosher D. S., Quignon P., Bustamante C. D., Sutter N. B., Mellersh C. S., Parker H.G., Ostrander E.A. (2007) A mutation in the myostatin gene increases muscle mass and enhances racing performance in heterozygote dogs. PLoS Genet 3, e79-.
|
 Oh Y. K., Kim J. P., Yoon H., Kim J. M., Yang J.S., Kim C.K. (2001) Prolonged organ retention and safety of plasmid DNA administered in polyethylenimine complexes. Gene Therapy 8, 1587-1592.
|
 Parker S. E., Borellini F., Wenk M. L., Hobart P., Hoffman S. L., Hedstrom R., Le T., Norman J.A. (1999) Plasmid DNA malaria vaccine: tissue distribution and safety studies in mice and rabbits. Human Gene Therapy 10, 741-758.
|
 Quezada A., Larson J., French M., Ponce R., Perrard J., Durland R., Coleman M. (2004) Biodistribution and safety studies of hDel-1 plasmid-based gene therapy in mouse and rabbit models. Journal of Pharmacy and Pharmacology 56, 177-185.
|
 Romero N. B., Braun S., Benveniste O., Leturcq F., Hogrel J. Y., Morris G. E., Barois A., Eymard B., Payan C., Ortega V., Boch A. L., Lejean L., Thioudellet C., Mourot B., Escot C., Choquel A., Recan D., Kaplan J. C., Dickson G., Klatzmann D., Molinier-Frenckel V., Guillet J. G., Squiban P., Herson S., Fardeau M. (2004) Phase I study of dystrophin plasmid-based gene therapy in Duchenne/Becker muscular dystrophy. Human Gene Therapy 15, 1065-1076.
|
 Schneider A.J., Friedmann T. (2006) Gene doping in sports: the science and ethics of genetically modified athletes. Advances in Genetics 51, 1-110.
|
 Schuelke M., Wagner K. R., Stolz L. E., Hubner C., Riebel T., Komen W., Braun T., Tobin J.F., Lee S.J. (2004) Myostatin mutation associated with gross muscle hypertrophy in a child. New England Journal of Medicine 350, 2682-2688.
|
 Sharp N.C. (2010) The human genome and sport, including epigenetics, gene doping, and athleticogenomics. Endocrinology and Metabolism Clinics of North America 39, 201-215.
|
 Shelton G.D., Engvall E. (2007) Gross muscle hypertrophy in whippet dogs is caused by a mutation in the myostatin gene. Neuromuscular Disorders 17, 721-722.
|
 Siriett V., Salerno M. S., Berry C., Nicholas G., Bower R., Kambadur R., Sharma M. (2007) Antagonism of myostatin enhances muscle regeneration during sarcopenia. Molecular Therapy 15, 1463-1470.
|
 Swatland H.J., Kieffer N.M. (1974) Fetal development of the double muscled condition in cattle. Journal of Animal Science 38, 752-757.
|
 Takei Y., Kadomatsu K., Yuzawa Y., Matsuo S., Muramatsu T. (2004) A small interfering RNA targeting vascular endothelial growth factor as cancer therapeutics. Cancer Research 64, 3365-3370.
|
 Thomas S. M., Zeng Q., Dyer K. F., Suscovich T. J., Kanter P. M., Whalen J. D., Watkins S.F., Grandis J.R. (2003) Tissue distribution of liposome-mediated epidermal growth factor receptor antisense gene therapy. Cancer Gene Therapy 10, 518-528.
|
 vanDeutekom J. C., Janson A. A., Ginjaar I. B., Frankhuizen W. S., Aartsma-Rus A., Bremmer-Bout M., den Dunnen J. T., Koop K., van der Kooi A. J., Goemans N. M., de Kimpe S. J., Ekhart P. F., Venneker E. H., Platenburg G. J., Verschuuren J. J., van Ommen G.J. (2007) Local dystrophin restoration with antisense oligonucleotide PRO051. New England Journal of Medicine 357, 2677-2686.
|
 Wells D.J. (2008) Gene doping: the hype and the reality. British Journal of Pharmacology 154, 623-631.
|
 West R.L. (1974) Red to white fiber ratios as an index of double muscling in beef cattle. Journal of Animal Science 38, 1165-1175.
|
 Whittemore L. A., Song K., Li X., Aghajanian J., Davies M., Girgenrath S., Hill J. J., Jalenak M., Kelley P., Knight A., Maylor R., O’Hara D., Pearson A., Quazi A., Ryerson S., Tan X. Y., Tomkinson K. N., Veldman G. M., Widom A., Wright J. F., Wudyka S., Zhao L., Wolfman N.M. (2003) Inhibition of myostatin in adult mice increases skeletal muscle mass and strength. Biochemical and Biophysical Research Communications 300, 965-971.
|
|
|
|
|
|
|