|
|
|
ABSTRACT |
The main purpose of this study was to determine the relative contributions of the aerobic and glycolytic systems during an incremental exercise test (IET). Ten male recreational long-distance runners performed an IET consisting of three-minute incremental stages on a treadmill. The fractions of the contributions of the aerobic and glycolytic systems were calculated for each stage based on the oxygen uptake and the oxygen energy equivalents derived by blood lactate accumulation, respectively. Total metabolic demand (WTOTAL) was considered as the sum of these two energy systems. The aerobic (WAER) and glycolytic (WGLYCOL) system contributions were expressed as a percentage of the WTOTAL. The results indicated that WAER (86-95%) was significantly higher than WGLYCOL (5-14%) throughout the IET (p < 0.05). In addition, there was no evidence of the sudden increase in WGLYCOL that has been previously reported to support to the “anaerobic threshold” concept. These data suggest that the aerobic metabolism is predominant throughout the IET and that energy system contributions undergo a slow transition from low to high intensity. |
Key words:
Oxygen uptake, blood lactate, aerobic threshold, anaerobic threshold, onset of blood lactate accumulation, energy metabolism contribution
|
Key
Points
- The aerobic metabolism contribution is the predominant throughout the maximal incremental test.
- The speed corresponding to the aerobic threshold can be considered the point in which aerobic metabolism reaches its maximal contribution.
- Glycolytic metabolism did not contribute largely to the energy expenditure at intensities above the anaerobic threshold.
|
Traditionally, some of the most important physiological predictors of long-distance running performance have been measured using incremental exercise tests (IET) (Costill et al., 1973; Fairshter et al., 1983; Saltin and Astrand, 1967). The IET is a standard procedure for determining maximal and submaximal variables such as maximal oxygen uptake (VO2max) (Gordon et al., 2011) and metabolic thresholds (Kindermann et al., 1979), respectively. After the classic study of Hill and Lupton, 1923, a large number of authors proposed that VO2max could be used as a gold standard for maximal aerobic power evaluation (Gordon et al., 2011; Hottenrott et al., 2012; Saltin et al., 1967; Taylor et al., 1955). However, although VO2max has been widely accepted as the upper limit of the body’s aerobic functioning (Day et al., 2003), it has also been suggested that this variable is not sensitive enough to reflect changes in endurance capacity (Edwards et al., 2003) and may not be the best predictor of endurance performance in homogenous groups of athletes (Conley et al., 1980; Noakes et al., 1990) or in untrained individuals (Yoshida et al., 1987). Consequently, other submaximal physiological variables should also be considered during IET to better predict endurance performance (Bentley et al., 2007; Edwards et al., 2003; Hagberg et al., 1983). It has been proposed that metabolic thresholds are useful physiological parameters to predict endurance performance because they reflect the adaptation from long-distance training (Edwards et al., 2003; Faude et al., 2009, Meyer et al., 2005). Historically, several methods have been proposed in the literature to determine these thresholds (see Svedahl and MacIntosh, 2003 for review). Previous studies have demonstrated that both aerobic (Hagberg and Coyle, 1983) and anaerobic (Svedenhag and Sjödin, 1984) thresholds are important parameters for success in long-distance events. It is believed that high aerobic and anaerobic thresholds may reflect the ability of athletes to tolerate very high velocities during long periods of exercise (Edwards et al., 2003; Meyer et al., 2005). In contrast, the terminologies utilized to discriminate metabolic thresholds may suggest an erroneous understanding about the profiles of metabolic pathways during an IET. As recently highlighted by Hopker et al., 2011, the term “anaerobic threshold” may not clearly indicate the moment at which the glycolytic system becomes the primary system resynthesizing ATP in exercised muscles. Although most sport scientists and coaches believe that metabolic pathways have a transitional nature, the profiles of aerobic and glycolytic metabolism during an IET have never been described in the literature. Understanding the relative aerobic and glycolytic contribution at each stage of an IET could provide a rational explanation for the human energy metabolism at a wide range of submaximal intensities. This information may lead to a more detailed understanding of the adaptations that result from the endurance training. Therefore, the main purpose of the current study was to estimate the glycolytic and aerobic contributions to performance during IET. Because the anaerobic threshold is considered the highest intensity that can be maintained without considerable blood lactate accumulation (Faude et al., 2009), it was hypothesized that the glycolytic system would increase its contribution to total energy expenditure at work rates above the anaerobic threshold.
Participants and proceduresTen male recreational long-distance runners (age 30 ± 4 years, body mass 75.7 ± 7.0 kg, height 1.78 ± 0.06 m, and body fat 15.2 ± 3.8%) volunteered to participate in this study. All subjects were healthy non-smokers with no cardiovascular or neuromuscular diseases. They were not taking regular medication or nutritional supplementation. They had competed regularly in regional competitions and been training for the last two years without interruption. The runners were involved in local 10 km competitions and had personal records of 40 ± 5 min. All runners performed only submaximal continuous aerobic training (50-70% VO2max) and had no previous high-intensity interval training or strength training experience. They were also familiar with exhaustive treadmill running. The participants received verbal explanations about the possible benefits and risks associated with the study and signed a written informed consent before participating in the study. The IET was conducted during the preparatory training period under normal laboratory conditions (temperature 22 ± 1 °C and relative humidity 50 ± 2%) at the same time of the day to avoid any possible diurnal variation effect on the performance. The subjects were instructed to refrain from exhaustive or unaccustomed exercises and the ingestion of caffeinated or alcoholic beverages for 24 hours before the tests. The procedures were in accordance with the Helsinki declaration of 1975, and the study was approved by the Ethics Committee for Human Studies of the School of Physical Education and Sport of the University of São Paulo.
Incremental exercise testBefore starting the IET, participants were asked to rest quietly in a standing position for five minutes to determine the baseline VO2 (VO2baseline). After a three-minute warm up at 6 km·h1, the speed was increased by 1.2 km·h1 every three minutes until volitional exhaustion (Heck et al., 1985). The treadmill (model TK35, CEFISE, Nova Odessa, Brazil) was set at a gradient of 1% throughout the IET to simulate outdoor running (Jones et al., 1996). A three-minute speed increment was chosen because it provides the most reliable and valid submaximal measurement of physiological variables (i.e., blood lactate and oxygen uptake) (Bentley et al., 2007). Subjects received strong verbal encouragement to continue as long as possible. Each stage was separated by a 10-second rest period, during which capillary blood samples were obtained from the earlobe (25 µL) and analyzed for blood lactate concentrations (YSI 1500 Sport, Yellow Springs Instruments, Yellow Springs, OH). Oxygen uptake (VO2) was measured breath-by-breath throughout the tests using a gas analyzer (Metalyzer 3b, Cortex, Leipzig, Germany). Calibration of the device was performed according to manufacturer specifications using ambient air, a gas of known composition containing 20.9% O2 and 5% CO2, and a 3 L syringe (Model 5530 Series, Hans Rudolph, Kansas City, USA). Heart rate was measured during the test with a heart rate monitor (S810, Polar Electro Oy, Kempele, Finland) that was coupled to the gas analyzer. The VO2max was determined when two or more of the following criteria were met: an increase in VO2 of less than 2.1 ml·kg1·min1 on two consecutive final stages, a respiratory exchange ratio greater than 1.1, a blood lactate concentration higher than 8.0 mmol·l1, and a heart rate ± 10 bpm of the maximal age-predicted heart rate (Howley et al., 1995). The speed corresponding to VO2max (Vmax) was established as the minimal speed that elicited VO2max (Bertuzzi et al., 2012). If a runner achieved VO2max during a stage that could not be maintained for one minute, the speed in the previous stage was defined as Vmax. The aerobic threshold was considered as the speed corresponding to blood lactate accumulation of 2 mmol·l1 as suggested by Kindermann et al., 1979, while the anaerobic threshold was the speed corresponding to 3.5 mmol·l1 of blood lactate accumulation as proposed by Heck et al., 1985. The highest speed achieved during the last completed stage was considered to be the peak treadmill velocity (Noakes et al., 1990). The maximal heart rate (HRmax) was defined as the highest value obtained at the end of the test.
Calculation of the energy system contributionsEnergy system contributions during each stage of the IET were determined as previously described (Bertuzzi et al., 2007; Bertuzzi et al., 2010). Briefly, the net aerobic energy system was estimated by subtracting VO2baseline from the VO2 exercise area integrated over time by the trapezoidal method. To estimate the glycolytic system contribution, a value of 1 mmol·l1 [La]net was considered to be equivalent to 3 ml O2·kg1 body mass (di Prampero and Ferretti, 1999). As the ATP-PCr system is predominantly used to resynthesize of ATP during high-intensity (3-5 times the power output that elicits O2max), short-duration exercises (~10 seconds) (Gastin, 2001), the contribution of alactic metabolism was not considered. The total metabolic demand (WTOTAL) was the sum of both energy metabolism contributions. The aerobic (WAER) and glycolytic (WGLYCOL) energy system contributions were also expressed as a percentage of the WTOTAL. A caloric equivalent of 20.9 kJ·l O21 was used for the energy systems calculations.
Statistical analysisThe distribution of the data was analyzed by the Shapiro-Wilk test, and the results showed a normal Gaussian distribution. The data are reported as the mean and standard deviation (SD). A paired t-test was used to compare the WAER and WGLYCOL contributions estimated during the IET. Repeated measure analyses of variance with Bonferroni correction for multiple comparisons were used to compare the WAER or WGLYCOL contribution among the stages of the IET. The level of significance was set at p < 0.05.
Table 1 shows the physiological and performance parameters measured during the IET. Table 2 shows the cardiorespiratory variables and blood lactate accumulation during the IET until 18 km·h1, which was the last completed stage. Lower values of minute ventilation (F(2,10) = 136.9, p < 0.05), blood lactate accumulation (F(2,10) = 19.3, p < 0.05), and heart rate (F(2,10) = 320.1, p < 0.05) measured during early stages (6-9.6 km·h1) were observed compared with those during more intense stages. No significant difference was found in the respiratory exchange ratio throughout the IET after Bonferroni correction for multiple comparisons (F(2,10) = 27.9, p > 0.05). Table 3 shows the absolute energy system contributions estimated for each stage. WAER was significantly higher when compared with WGLYCOL throughout the IET (p < 0.05). The WAER increased continuously up to 183.9 ± 23.5 kJ, while WGLYCOL increased continuously up to 30.6 ± 3.0 kJ. However, this increase was not statistically significant in both WAER (F(2,10) = 319.6) and WGLYCOL (F(2,10) = 23.6) after Bonferroni correction for multiple comparisons (p > 0.05). Figure 1 shows the energy system interaction ex-pressed as percentage of WTOTAL. The relative WAER contribution was statistically higher than WGLYCOL during all the stages of the IET (p < 0.05). The aerobic contribution decreased continuously from 95.7 ± 1.5% at 6 km·h1 to 86.1 ± 4.7% at 18 km·h1, while the WGLYCOL increased continuously from 5.3 ± 1.5% to 13.9 ± 4.7%. However, this increase in relative WGLYCOL (F(2,10) = 11.7) and reduction in relative WAER (F(2,10) = 11.5) were not statistically significant throughout the IET after Bonferroni correction for multiple comparisons (p > 0.05).
It has been recently emphasized that the terminologies utilized to describe metabolic threshold concepts may introduce an erroneous understanding about metabolic pathway profiles during the IET, especially because the term “anaerobic threshold” may not clearly indicate the predominance of the glycolytic energy system in ATP resynthesis (Hopker et al., 2011). It is known that both pyruvate and lactate are the end products of the anaerobic glycolysis. Lactate formation via enzyme lactate dehydrogenase is caused by a local mass action of high pyruvate and NADH concentrations. This suggests that high lactate concentrations detected in the blood could indicate the predominance of the glycolytic pathway. However, comparisons between WAER and WGLYCOL during graded incremental exercise tests had not been reported in the literature yet. The results of the present study revealed that the relative WAER progressively decreased by only ~9% from the first to the last stage, suggesting that aerobic metabolism is predominant throughout IET. In addition, no evidence was observed of a sudden increase in glycolytic contribution during the IET, which is the basis of the “anaerobic threshold” concept. Aerobic threshold has been considered to be the upper limit of a nearly exclusive aerobic metabolism based mainly on the blood lactate concentration (Kindermann et al., 1979) and gas exchange (Meyer et al., 2005) responses. In the present study, we examined the WAER and WGLYCOL using the oxygen uptake area and oxygen equivalent for blood lactate accumulation, respectively. This approach allowed a direct comparison between the metabolism pathway contributions during the IET. Our results support previous findings in which the highest aerobic contribution was found at the speed corresponding to the aerobic threshold (Faude et al., 2009). In addition, although no significant difference was found between the contributions of both energy pathways throughout the IET, the speed corresponding to the aerobic threshold was the last stage before the initiation of a slow consistent decrease in the relative WAER (Figure 1). Despite the fact that substrate oxidation was not determined in the present study, this may be related to the fat oxidation that occurs in exercises with low intensities. Previous findings have suggested a non-significant difference between the intensity corresponding to the aerobic threshold and the intensity in which the highest rate of fat oxidation is elicited in healthy men (Achten and Jeukendrup, 2004). It has been proposed that the first rise in lactate concentration occurs at the same intensity in which maximal fat oxidation rate is elicited. This occurs because the increased glycolytic flux appears to inhibit the long-chain free fatty acid entry in the mitochondria (Achten and Jeukendrup, 2004). Thus, the highest relative WAER observed at the aerobic threshold may reflect the oxygen required for the fat oxidation by slow twitch fibers in moderately trained long-distance runners. Notably, this highest relative WAER observed at aerobic threshold can reflect the optimal stimulus to produce the adaptations related to endurance performance. For instance, Seiler and Kjerland, 2006 analyzed the intensities chosen by highly trained cross-country skiers (VO2max ~73 ml·kg1·min1) during a pre-competition preparation period using a three intensity-zone model. It was observed that the athletes performed 75%, 5-10%, and 15-20% of the total training duration at intensities corresponding to the aerobic threshold, between the aerobic and anaerobic thresholds, and above the anaerobic threshold, respectively. These findings demonstrated that long-distance athletes preferentially train at intensities close to the aerobic threshold. According to Seiler and Kjerland, 2006, the training time spent at intensities slightly below the aerobic threshold is an important source of stress, which generates a high-oxidative flux in the working muscles during multiple daily sessions. In contrast to our hypothesis, there was no significant rise in WGLYCOL at intensities between the anaerobic threshold and VO2max. Some studies have emphasized the importance of the utilized terminologies to discriminate between metabolic thresholds (Myers and Ashley, 1997). Specifically, it was suggested that the term “anaerobic threshold” may not clearly indicate that the WGLYCOL becomes predominantly utilized for ATP resynthesis in exercising muscles at intensities above the anaerobic threshold (Hopker et al., 2011). Similar to the aerobic threshold concept, the anaerobic threshold concept was proposed based only on the blood lactate responses during the IET (Meyer et al., 2005). To date, comparisons between WAER and WGLYCOL at or above the threshold have not been reported. Our data have revealed that WAER and WGLYCOL at the anaerobic threshold corresponded to ~93% and ~7% of total metabolic demand, respectively. This means that despite increased WGLYCOL at intensities at or above the anaerobic threshold, the WAER was predominant during all stages of the IET. Therefore, the ability of the anaerobic threshold to predict endurance performance may not be directly (i.e., ATP resynthesis) or indirectly (i.e., metabolite accumulation) linked to glycolytic metabolism. Data from the current study provide a rational basis for the interactions between energy metabolism systems at a wide range of submaximal intensities. It was found that WAER contributed ~95% and ~86% to the total energy expenditure at the start and end of IET, respectively. This corroborates previous findings suggesting that WGLYCOL has a significant contribution only at intensities above VO2max. Using the maximal accumulated oxygen deficit method, Mezzani et al., 2008 reported that in patients with left ventricular dysfunction and healthy subjects, WGLYCOL contributed ~35% and 27% of the total energy expenditure at intensities corresponding to 133% and 121% of VO2max, respectively. Utilizing a similar method, Spencer and Gastin, 2001 showed that in highly trained athletes, the relative contributions of the WGLYCOL at running events were ~71, ~57, ~44, and ~16% at intensities corresponding to ~200, ~150, ~113, and ~103% of VO2max, respectively. Therefore, our results complement a growing body of work demonstrating that WGLYCOL is responsible for a negligible contribution to the total energy expenditure at intensities at and below VO2max. Some potential limitations should be considered when interpreting the current findings. Previous studies have reported restrictions related to the usage of VO2 and [La] to represent aerobic and glycolytic metabolism, respectively (Gastin, 1994; Gladden, 2004; Green and Dawsond 1993). It has been suggested the determination of the energy pathway contributions of active muscles through the assessment of whole-body physiological variables can be inaccurate. For example, the appearance of slow component of VO2 would overestimate the WAER contribution (Mezzani et al., 2008). However, it is possible that this had no influence on our calculations because the three-minute stage duration was too short to allow the slow component to appear. Regarding the determination of the glycolytic metabolism contribution, it is relevant to notice that lactate has been considered an important carbohydrate fuel source during exercise (Gladden, 2008, Gladden, 2004). Muscle lactate oxidation in skeletal muscle mitochondria involves a sequential mechanism in which protons and lactate molecules bind to the monocarboxylate transporter 1 (MCT1). Upon the start of the moderate intensity exercise, lactate and pyruvate concentrations increase at low levels without changing lactate/pyruvate ratio until a threshold at which lactate abruptly increases without pyruvate changes (Wasserman et al., 1985). Because of its higher concentration compared to pyruvate, muscle lactate would be transported across the inner mitochondrial membrane by MCT1 (Gladden, 2008). Consequently, muscle lactate may be removed by a cell-to-cell lactate shuttle and used as an energy fuel by oxidative muscle fibers before it escapes into circulation. As a result, the estimated value of the glycolytic metabolism contribution based on blood lactate accumulation could be underestimated, especially at intensities above the anaerobic threshold. However, it has been showed that the lactate/pyruvate ratio increases in a similar manner as the VO2 responses during the IET (Wasserman et al., 1985). Consequently, the 3 ml O2·kg1·mmol1 lactate·L1 equivalent could be used as an empirical method that allows us to estimate the glycolytic energy release during dynamic exercise. Alternatively, intramuscular metabolites could be used to quantify the glycolytic contribution during the IET. However, the muscle tissue used for these measurements is usually obtained from small muscle mass. Consequently, it would be necessary to determine the muscle mass that is involved in a specific exercise, which could result in an inaccurate representation of glycolytic metabolism activation (Gastin, 1994). In addition, the muscle biopsy technique requires specialized personnel, and athletes are often uncomfortable participating in studies that use this procedure, especially when many biopsies are required.
Data from the present study demonstrate that the highest WAER as well as the beginning of the aerobic/glycolytic transition occurs at a speed close to the aerobic threshold. In addition, WAER and WGLYCOL demonstrate a very slow progressive transition pattern throughout the IET, without the sudden increase in WGLYCOL espoused by the “anaerobic threshold” concept.
|
AUTHOR BIOGRAPHY |
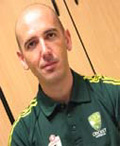 |
Rômulo Bertuzzi |
Employment: Associate professor, Department of Sport, School of Physical Education and Sport, São Paulo University, São Paulo, Brazil. |
Degree: PhD |
Research interests: Physiological determinants of endurance performance and quantification of energetic systems contribution during exercise. |
E-mail: bertuzzi@usp.br |
|
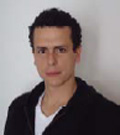 |
Eduardo M.F. Nascimento |
Employment: Master student, Department of Sport, School of Physical Education and Sport, São Paulo University, São Paulo, Brazil. |
Degree: MSc |
Research interests: Metabolism and quantification of energetic systems contribution during exercise. |
E-mail: eduardomarcel@usp.br |
|
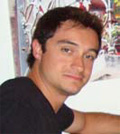 |
Rodrigo P. Urso |
Employment: Master student, Department of Sport, School of Physical Education and Sport, São Paulo University, São Paulo, Brazil. |
Degree: Graduate in Sport Science |
Research interests: Metabolism and quantification of energetic systems contribution during exercise. |
E-mail: rodrigo.urso@usp.br |
|
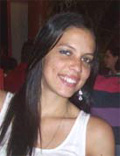 |
Mayara Damasceno |
Employment: Master student, Department of Sport, School of Physical Education and Sport, São Paulo University, São Paulo, Brazil. |
Degree: Graduate in Physical Education |
Research interests: Pacing strategy, strength training and endurance performance. |
E-mail: damascenomay@usp.br |
|
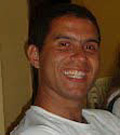 |
Adriano E. Lima-Silva |
Employment: Adjunct Professor of Federal University of Alagoas, Maceió, Brazil. |
Degree: Dr |
Research interests: Pacing strategy, metabolism and quantification of energetic systems contribution during exercise. |
E-mail: adrianosilva@usp.br |
|
|
|
REFERENCES |
 Achten J., Jeukendrup A.E. (2004) Relation between plasma lactate concentration and fat oxidation rates over a wide range of exercise intensities. International Journal Sports Medicine 25, 32-37.
|
 Bentley D.J., Newell J., Bishop D. (2007) Incremental exercise test design and analysis: implications for performance diagnostics in endurance athletes. Sports Medicine 37, 575-586.
|
 Bertuzzi R., Bueno S., Pasqua L.A., Acquesta F.M., Batista M.B., Roschel H., Kiss M.A., Serrão J.C., Tricoli V., Ugrinowitsch C. (2012) Bioenergetics and neuromuscular determinants of the time to exhaustion at velocity corresponding to VOmax in recreational long-distance runners. Journal of Strength and Conditioning Research 26, 2096-2102.
|
 Bertuzzi R.C., Franchini E., Kokubun E., Kiss M.A. (2007) Energy system contributions in indoor rock climbing. European Journal or Applied Physiology 101, 293-300.
|
 Bertuzzi R.C., Franchini E., Ugrinowitsch C., Kokubun E., Lima-Silva A.E., Pires F.O., Nakamura F.Y., Kiss M.A. (2010) Predicting MAOD using only a supramaximal exhaustive test. International Journal of Sports Medicine 31, 477-481.
|
 Conley D.L., Krahenbuhl G.S. (1980) Running economy and distance running performance of highly trained athletes. Medicine and Science in Sports and Exercise 12, 357-360.
|
 Costill D.L., Thomason H., Roberts E. (1973) Fractional utilization of the aerobic capacity during distance running. Medicine and Science in Sports 5, 248-252.
|
 Day J.R., Rossiter H.B., Coats E.M., Skasick A., Whipp B.J. (2003) The maximally attainable VO during exercise in humans: the peak vs. maximum issue. Journal of Applied Physiology 95, 1901-1907.
|
 di Prampero P.E., Ferretti G. (1999) The energetics of anaerobic muscle metabolism: a reappraisal of older and recent concepts. Respiratory Physiology 118, 103-115.
|
 Edwards A.M., Clark N., MacFadyen A.M. (2003) Lactate and ventilatory thresholds reflect the training status of professional soccer players where maximum aerobic power is unchanged. Journal of Sports Science and Medicine 2, 23-29.
|
 Fairshter R.D., Walters J., Salness K., Fox M., Minh V.D., Wilson A.F. (1983) A comparison of incremental exercise tests during cycle and treadmill ergometry. Medicine and Science in Sports and Exercise 15, 549-554.
|
 Faude O., Kindermann W., Meyer T. (2009) Lactate threshold concepts: How valid are they?. Sports Medicine 39, 469-490.
|
 Gastin P.B. (1994) Quantification of anaerobic capacity. Scandinavian Journal of Medicine Science and Sports 4, 91-112.
|
 Gastin P.B. (2001) Energy system interaction and relative contribution during maximal exercise. Sports Medicine 31, 725-741.
|
 Gladden L.B. (2004) Lactate metabolism: a new paradigm for the third millennium. Journal of Physiology 558, 5-30.
|
 Gladden L.B. (2008) A “Lactatic” perspective on metabolism. Medicine and Science in Sports and Exercise 40, 477-485.
|
 Gordon D., Hopkins S., King C., Keiller D., Barnes R.J. (2011) Incidence of the plateau at VOmax is dependent on the anaerobic capacity. International Journal of Sports Medicine 32, 1-6.
|
 Green S., Dawsond B. (1993) Measurement of anaerobic capacities in humans. Definitions, limitations and unsolved problems. Sports Medicine 15, 312-327.
|
 Hagberg J.M., Coyle E.F. (1983) Physiological determinants of endurance performance as studied in competitive racewalkers. Medicine and Science in Sports and Exercise 15, 287-289.
|
 Heck H., Mader A., Hess G., Mücke S., Müller R., Hollmann W. (1985) Justification of the 4-mmol/l lactate threshold. International Journal of Sports Medicine 6, 117-130.
|
 Hill A.V, Lupton H. (1923) Muscular exercise, lactic acid, and the supply and utilization of oxygen. Quarterly Journal of Medicine 16, 135-171.
|
 Hopker J.G., Jobson S.A., Pandit J.J. (2011) Controversies in the physiological basis of the †§anaerobic threshold†§ and their implications for clinical cardiopulmonary exercise testing. Anaesthesia 66, 111-123.
|
 Hottenrott K., Ludyga S., Schulze S. (2012) Effects of high intensity training and continuous endurance training on aerobic capacity and body composition in recreationally active runners. Journal of Sports Science and Medicine 11, 483-488.
|
 Howley E.T., Bassett D.R., Welch H.G. (1995) Criteria for maximal oxygen uptake: review and commentary. Medicine and Science in Sports and Exercise 27, 1292-1301.
|
 Jones A.M., Doust J.H. (1996) A 1% treadmill grade most accurately reflects the energetic cost of outdoor running. Journal of Sports Science 14, 321-327.
|
 Kindermann W., Simon G., Keul J. (1979) The significance of the aerobic-anaerobic transition for the determination of work load intensities during endurance training. European Journal of Applied Physiology and Occupation Physiology 42, 25-34.
|
 Meyer T., Lucía A., Earnest C.P., Kindermann W. (2005) A conceptual framework for performance diagnosis and training prescription from submaximal gas exchange parameters-theory and application. International Journal of Sports Medicine 26, S38-48.
|
 Mezzani A., Corrà U., Andriani C., Giordano A., Colombo R., Gianuzzi P. (2008) Anaerobic and aerobic relative contribution to total energy release during supramaximal effort in patients with left ventricular dysfunction. Journal of Applied Physiology 104, 97-102.
|
 Myers J., Ashley E. (1997) Dangerous curves. A perspective on exercise, lactate, and the anaerobic threshold. Chest 111, 787-795.
|
 Noakes T.D., Myburgh K.H., Schall R. (1990) Peak treadmill running velocity during the VO max test predicts running performance. Journal of Sports Science 8, 35-45.
|
 Saltin B., Astrand P.O. (1967) Maximal oxygen uptake in athletes. Journal Applied of Physiology 23, 353-358.
|
 Seiler K.S., Kjerland G.O. (2006) Quantifying training intensity distribution in elite endurance athletes: is there evidence for an "optimal" distribution?. Scandinavian Journal of Medicine Science and Sports 16, 49-56.
|
 Spencer M. R., Gastin P. B. (2001) Energy system contribution during 100- to 1500-m in highly trained athletes. Medicine and Science in Sports and Exercise 33, 157-162.
|
 Svedahl K., MacIntosh B.R. (2003) Anaerobic threshold: the concept and methods of measurement. Canadian Journal of Applied Physiology 28, 299-323.
|
 Svedenhag J., Sjödin B. (1984) Maximal and submaximal oxygen uptakes and blood lactate levels in elite male middle- and long-distance runners. International Journal of Sports Medicine 5, 255-261.
|
 Taylor H.L., Buskirk E., Henschel A. (1955) Maximal oxygen uptake as an objective measure of cardiorespiratory performance. Journal of Applied Physiology 8, 73-80.
|
 Wasserman K., Beaver W., Davis J.A., Pu J.Z., Heber D., Whipp B.J. (1985) Lactate, pyruvate, and lactate-to-pyruvate ratio during exercise and recovery. Journal of Applied Physiology 59, 935-940.
|
 Yoshida T., Chida M., Ichioka M., Suda Y. (1987) Blood lactate parameters related to aerobic capacity and endurance performance. European Journal of Applied Physiology and Occupation Physiology 56, 7-11.
|
|
|
|
|
|
|